- Department of Endocrinology and Metabolic Diseases, Dalian University Affiliated Xinhua Hospital, Dalian, Liaoning, China
As a new way of programmed cell death, pyroptosis plays a vital role in many diseases. In recent years, the relationship between pyroptosis and type 2 diabetes (T2D) has received increasing attention. Although the current treatment options for T2D are abundant, the occurrence and development of T2D appear to continue, and the poor prognosis and high mortality of patients with T2D remain a considerable burden in the global health system. Numerous studies have shown that pyroptosis mediated by the NLRP3 inflammasome can affect the progression of T2D and its complications; targeting the NLRP3 inflammasome has potential therapeutic effects. In this review, we described the molecular mechanism of pyroptosis more comprehensively, discussed the most updated progress of pyroptosis mediated by NLRP3 inflammasome in T2D and its complications, and listed some drugs and agents with potential anti-pyroptosis effects. Based on the available evidence, exploring more mechanisms of the NLRP3 inflammasome pathway may bring more options and benefits for preventing and treating T2D and drug development.
Introduction
T2D is a multifactorial autoimmune disease characterized by glucose and lipid metabolism disturbances, insulin resistance (IR), and absolute or relative insulin deficiency (1, 2). In addition, chronic inflammation runs through the entire process of the development of diabetes and its complications (3–5), exacerbating metabolic imbalances and the development of complications (6, 7). Worldwide, the incidence of T2D is still increasing (8, 9), and its complications remain a significant cause of death (10). Inflammatory response and metabolic disorders are a vicious cycle in type 2 diabetes, and chronic inflammation, blood sugar, and lipid disorders promote each other (11–13). A better understanding of the inflammatory response may have a significant effect on the treatment and outcome of T2D.
As a form of programmed cell death, pyroptosis is essential in maintaining physiology homeostasis and pathogen invasion (14). With the discovery of gasdermins family, the scope of pyroptosis has expanded. The earliest study of pyroptosis dates back to 1986, Friedlander’s study of mouse macrophage death and content release (15). It was not until 2001 that D’Souza et al. coined the term pyroptosis to describe inflammatory programmed cell death (16). In 2015, pyroptosis was defined as the inflammatory programmed cell death mediated by gasdermins (17). The gasdermins family includes gasdermin A/B/C/D (GSDMA/B/C/D), gasdermin E (GSDME, also known as DFNA5), and DFNB59 (Pejvakin, PJVK) (18). When pyroptosis occurs, gasdermins are cleaved by caspases into two fragments (the N-terminal pore-forming domain (PFD) and the C-terminal repressor domain (RD)); the N-terminal PFD oligomerizes and forms pores in the cell membrane, leading to the release of inflammatory factors and caspases, which promote cell pyroptotic death (18–20). For a long time, pyroptosis was thought to be caspase-1-induced monocyte death, and now the definition of pyroptosis has been expanded to include caspase-4/5/11 (21). Current research suggests that caspase-1 and caspase-4/5/11 are only related to pyroptosis. In contrast, caspase-2, caspase-7, and caspase-10 are only associated with apoptosis (22–26). Other caspases such as caspase-3/8/9 are involved in the process of pyroptosis and apoptosis (17, 27–32), and play an essential role in the occurrence and development of innate immune diseases, autoimmune diseases and tumors (33–37). Previous studies suggested that Caspase-3 was an executor of apoptosis, but a recent study found that Caspase-3 can induce pyroptosis by cleaving GSDME (27, 38). Apoptosis-related protein caspase-8 can also directly cleave GSDMC and GSDMD to induce pyroptosis (28, 39, 40). In addition, caspase-9 is also involved in pyroptosis by activating caspase-3 (41) (Figure 1). With the deepening of research, the mechanism of pyroptosis has gradually become apparent, and the relationship between pyroptosis and disease has also been more explored and studied.
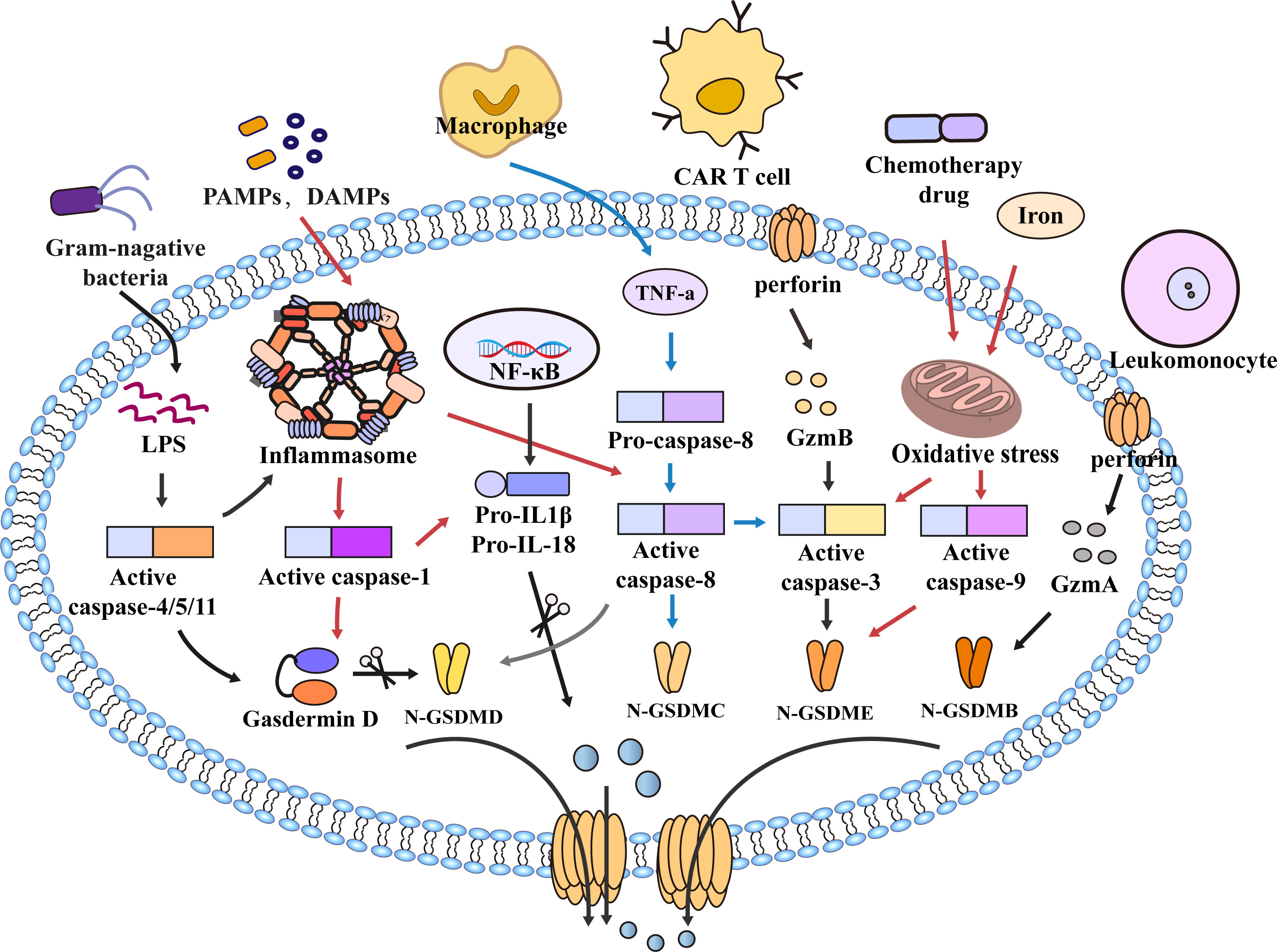
Figure 1 Molecular mechanism of pyroptosis. In the caspase-1-dependent pathway, PAMPs and DAMPs mediate inflammasome assembly and activate caspase-1, which cleaves GSDMD and pro-IL-1β/18. N-GSDMD forms non-selective pores on the cell membrane surface, and IL-1β and IL-18 are secreted out of the cell membrane through the pores formed by N-GSDMD, which further leads to cell lysis and death. In the caspase-1-independent pathway, LPS of bacteria activates caspase-4/5/11 and cleaves GSDMD to trigger pyroptosis. However, caspase-4/5/11 can also activate inflammasome assembly and caspase-1 to induce pyroptosis. In addition, in the caspase-8-mediated pathway, TNF-α induces the activation of caspase-8, cleavage of GSDMD leads to pyroptosis, and caspase-8 can also cleave GSDMC and GSDME, Furthermore, the NLRP3 inflammasome also activate Caspase-8. Granzyme also mediates pyroptosis, such as GzmB released from CAR T cells induces pyroptosis by activating caspase-3 and cleaving GSDME. In the caspase-9-mediated pathway, chemotherapy drugs and iron cause mitochondrial stress, activate caspase-3/9, and cleave GSDMB/E to mediate pyroptosis. Furthermore, GzmA in cytotoxic leukomonocyte enters target cells via perforin and cleaves GSDMB resulting in cell pyroptotic death. Similarly, GSDMB/C/E also forms pores to mediate the secretion of inflammatory mediators out of the membrane, amplify the inflammatory response, and promote cell death.
Recent studies have shown that pyroptosis, especially the NLRP3 inflammasome-mediated pyroptosis, plays a vital role in the progression of diabetes and its complications (42). However, the relationship between diabetes and pyroptosis has not been fully understood, and the proportion of progression in diabetes is not known. Although some articles on similar topics have been published in recent years (42), in this review, we will mainly discuss the current progress of pyroptosis and summarize the connection between pyroptosis mediated by NLRP3 and T2D and its complications more comprehensively. In addition, we will highlight some current therapeutic strategies targeting the NLRP3 inflammasome signaling pathway, which may provide new targets for treating diabetes.
Molecular mechanism of pyroptosis
Canonical pyroptosis
The classical pyroptotic pathway mediates caspase-1 activation through inflammasome assembly, triggering the cleavage of GSDMD and the release of inflammatory substances such as IL-1β and IL-18 into the extracellular space, leading to further expansion of the inflammatory response (43–45). The inflammasome is a complex of multiple molecules that begins to assemble through recognizing danger signals by cytosolic pattern recognition receptors (PRRs) (46). PRRs, also known as inflammasome sensors, include NLRP1, NLRP3, NLRC4, AIM2, and pyrin (47, 48). Most inflammasomes are composed of three functional domains: NOD-like receptors (NLRs) of inflammatory sensors, the apoptosis-associated speck-like protein containing a caspase recruitment domain (CARD) (ASC) and caspase (49–51). Whether the N-terminus of NLRs contains CARD or Pyrin domain (PYD), NLRs are divided into NLRPs or NRCs. The N-terminus of NLRCs has one or more CARD domains, such as NLRC4; the N-terminus of NLRPs is PYD, such as NLRP1 (containing PYD and CARD domains) and NLRP3 (52–56). Unlike NLRs, ASCs have both PYD and CARD domains (53). The inflammatory sensors AIM2 and pyrin are also involved in inflammasome assembly despite lacking NLRs. AIM2 consists of a C-terminal HIN-200 domain and an N-terminal PYD fragment (57–60). However, pyrin comprises an N-terminal PYD, two B-boxes, a coiled part, and a C-terminal B30.2 domain (also known as SPRY/PRY domain). Mouse Pyrin lacks the C-terminal B30.2 domain but is functionally similar to humans (53, 61, 62).
Many microbial infections, as well as non-microbial diseases, can cause inflammasome activation, for example, Val-boroPro (Talabostat, PT-100) (63) Toxoplasma gondii (55), Bacillus anthracis and its anthrax lethal toxin (64, 65) can cause the activation of NLRP1. NLRP3 can recognize pathogen-associated molecular patterns (PAMPs) and danger-associated molecular patterns (DAMPs), infectious microbes or their evolutionarily conserved molecular patterns are called PAMPs. After the body is infected, an inflammatory response is induced to cause damage. DAMPs are immunostimulatory molecular patterns in sterile inflammation and are positively correlated with damage. DAMPs are released when tissue is damaged and initiate an inflammatory response (66). Such as advanced glycation end products (AGEs), high mobility protein (HMGB-1), S100 protein family, heat shock protein family (HSPs), toxins, extracellular matrix (ECM), nucleic acids and ATP (67–70), induce inflammasome activation with the involvement of the kinase NEK7 (71–73). NLRC4 is activated by the binding of NAIP proteins and ligands (flagellin and type 3 secretion system (T3SS) proteins) (74–76). AIM2 is activated by combining the C-terminal HIN-200 domain with cytoplasmic dsDNA (57–60, 77). Another inflammatory sensor, pyrin, is induced to activate and assemble through small GTPases of the Rho family (61, 78, 79). Rho-inactivating toxins (Clostridium difficile glycosyltransferase TcdB, Vibrio parahaemolyticus VopS) and the Yersinia pestis GTPase-activating protein (YopE) and cysteine protease (YopT) can also induce pyrin activation (80, 81). Activating these sensors results in oligomerization, which forms an inflammasome complex by recruiting ASC and caspase1. Interestingly, the inflammatory sensors NLRP1 and NLRC4, due to the CARD domain at the N-terminus, do not require the recruitment of ASCs to activate caspase-1 directly (53). Interestingly, inflammatory sensors NLRP1 and NLRC4, due to their N-terminal containing CARD domains, can directly activate caspase-1 without recruiting ASC. After inflammasome assembly, caspase-1 is hydrolyzed into two fragments (82); On the one hand, activated caspase-1 hydrolyzes GSDMD into a C-terminus of 22 kDa (C-GSDMD) and an N-terminus of 31 kDa (N-GSDMD). N-GSDMD can form pores in cell membranes (83, 84). On the other hand, caspase-1 cleaves pro-IL-1β/18 into mature IL1β and IL-18, which are released extracellularly through pores formed by GSDMD, resulting in cell pyroptotic death (17–19, 85, 86) (Figure 2).
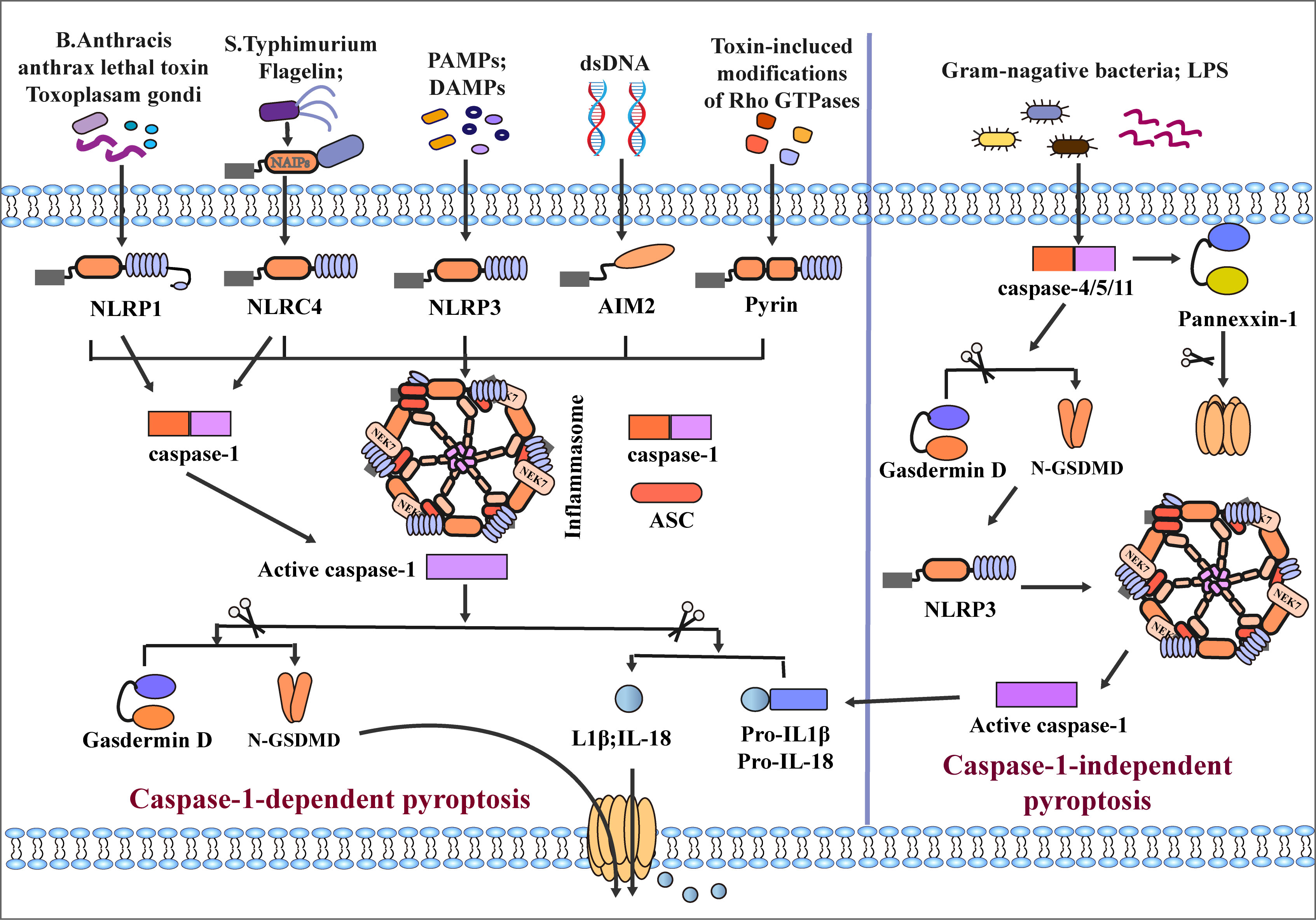
Figure 2 Classical and non-classical inflammasome assembly. The canonical pathway is mediated by caspase-1. When NLRP1, NLRC4, NLRP3, AIM, and Pyrin inflammatory sensors receive different stimuli, recruiting ASC and caspase-1 to mediate the assembly of inflammasomes and activate caspase-1. Among them, the assembly of NLRP3 and NLRC4 inflammasomes requires the participation of NEK7 and NAIPs, respectively, and NLRP1 and NLRC4 can directly activate case page-1 due to their CARD domains. After activation of Caspase-1, GSDMD and pro-IL-1β/18 is cleaved, and the pore formed by GSDMD on the cell membrane surface mediates secretion of IL-1β/18 out of the cell and induces pyroptosis. The non-canonical pathway is mediated by caspase-4/5/11; LPS of Gram-negative bacteria directly induces caspase-4/5/11 activation and cleaves GSDMD and Pannexin-1. N-GSDMD induces caspase-1 activation by activating NLRP3 inflammasome assembly, which mediates the release of inflammatory mediators and pyroptosis.
Non-canonical pyroptosis
In the caspase-1-independent pyroptosis pathway, human caspase-4/5 or mouse caspase-11 can be directly activated by N-terminal CARD combined with lipopolysaccharide (LPS) (87–89). After activation of caspase-4/5/11, GSDMD is cleaved into C/N-GSDMD, N-GSDMD is transferred to the cell membrane to form plasma membrane pores, and the assembly of NLRP3 inflammasome is initiated at the same time (90). However, in the caspase-1-independent pathway, many studies suggested that caspase-4/5/11 cannot directly cleave pro-IL-1β/18, which requires activation of the NLRP3/caspase-1 pathway through N-GSDMD to induce induction Maturation and secretion of IL-1β/IL-18 (31, 91–93). Some studies have also found that caspase-4 can directly cleave pro-IL-1β and pro-IL-18 (94). In addition, Yang et al. found that caspase-11 can specifically cleave Pannexin-1, causing the release of ATP (95). Meanwhile, cleavage of GSDMD by caspase-4/5/11 leads to the efflux of K+ and IL-1β/IL-18, eventually leading to pyroptosis (17, 92) (Figure 2). It is worth noting that Guanylate-binding proteins (GBPs) play an important role in non-canonical pyroptosis. GBPs could protect against bacterial infection, when cells are infected with LPS, GBPs would contribute to LPS release into the cytosol and activation of the noncanonical caspase-11 inflammasome, GBPs contribute to secretion of IL-1βand IL-18, and induction of pyroptosis (96, 97).
Other signaling pathways
Traditionally, caspase-3 mainly promotes the occurrence of apoptosis. However, recent studies have found that activation of caspase-3 can cleave GSDME into N/C-terminal fragments. Similar to N-GSDMD, N-GSDME can cause the formation of pores in the cell membrane and promote cell pyroptotic death (38, 98). In addition, Orning and Sarhan et al. found that Yersinia induced activation of caspase-8 after infection of mouse macrophages, resulting in the lysis of GSDMD (28, 29). Wu Qiao et al. found that metabolite α-KG can mediate pyroptosis of Hela cells via caspase-8/GSDMC (99). Hou et al. also proposed that PD-L1 mediates caspase-8/GSDMC activation in breast cancer cells, resulting in pyroptotic death of breast cancer cells (39); Jiang et al. reported that caspase-1, 3, and 7 could mediate the cleavage of GSDME leading to pyroptosis (100). Furthermore, Chemotherapeutic drugs, iron also mediate the activation of the caspase-9/GSDME/caspase-3 axis to induce pyroptosis. Interestingly, recent studies have found that the Granzyme family can cause pyroptosis (100). Zhang Z et al. found that GzmB can directly cleave GSDME to induce pyroptosis and inhibit tumor growth (101). Similarly, Liu Y et al. found that chimeric antigen receptor (CAR) T cells can activate GSDME to induce pyroptosis (102). Zhou Z et al. reported that GzmA derived by leukomonocyte induces pyroptosis by cleaving GSDMB at Lys229/Lys244 sites (103) (Figure 2).
NLRP3 inflammasome-mediated pyroptosis and type 2 diabetes
NOD-like receptor (NLR) family pyrin domain-containing 3 (NLRP3) is an important PRR in the cytoplasm. The NLRP3 inflammasome consists of a sensor (NLRP3), an aptamer (ASC), and an effector (caspase 1). The NLRP3 inflammatory sensor is composed of a C-terminal leucine-rich repeat (LRR), a nucleotide-binding and oligomerization domain (NACHT), and an N-terminal PYD domain that recruits caspases (104). Activation of the NLRP3 inflammasome involves two signals, the first signal is the initiation of NLRP3 gene transcription mediated by the NF-κB pathway, and the second signal is the activation of NLRP3 inflammasome sensors (105). The NLRP3 sensor self-oligomerizes through homotypic NACHT domain interactions. Oligomeric NLRP3 recruits ASCs through homotypic PYD-PYD domain interactions and induces ASC aggregation into a macromolecular focal point called the ASC speck. Subsequently, the assembled ASCs recruit proaspase-1 through homotypic CARD–CARD domain interactions to form the NLRP3-ASC-caspase-1 protein complex which is known as the NLRP3 inflammasome (106).
In type 2 diabetes, A variety of metabolites and factors such as glucose and fatty acids (107), LPS released by the gut microbiota (108, 109), mitochondrial reactive oxygen species (mROS) (110), the international association for preventive pediatrics(IAPP) (111), ceramide (112), amino acid homocysteine (113) and ATP (114) can activate the NLRP3 inflammasome. After stimulation, the NLRP3 inflammasome is activated with the participation of the ligand NIMA-related kinase 7 (NEK7) (71–73).
In 2010, Tschopp et al. first proposed that the NLRP3 inflammasome may be involved in the progression of T2DM (115). Subsequent studies have shown that NLRP3 inflammasome activation can aggravate IR and lead to further damage to islet β cells and promote T2D progression (5, 112, 116–119); some recent studies have also demonstrated that the activation of the NLRP3 inflammasome mediates the pyroptosis of pancreatic β cells (108, 120); for example, in the study of Yuan, J et al., the use of the NLRP3 inhibitor MCC950 ameliorated islet cell damage (121). Furthermore, pyroptosis mediated by the NLPR3 inflammasome plays a vital role in the progression of various complications such as diabetic nephropathy (DN) and diabetic cardiomyopathy (DCM) (Figure 3) (122–125). Recent studies have shown the potential therapeutic role of the NLRP3 inflammasome/pyroptosis signaling pathway in type 2 diabetes and its complications.
Microvascular complications
Diabetic nephropathy
As one of the microvascular complications of diabetes, DN is considered a sterile inflammatory disease and the leading cause of death in end-stage renal disease and patients with type 2 diabetes (126). Pyroptosis mediated by the NLRP3 inflammasome plays an important role in the development of diabetic nephropathy (122, 127, 128). In a previous study, Shahzad K et al. found that activation of the NLRP3 inflammasome in non-myeloid-derived cells exacerbates DN (127). A recent study showed that the expression levels of pyroptosis-related proteins such as NLRP3, caspase-1, and IL-1β were significantly increased in STZ-treated diabetic rats (129). An X et al. also found that in the HFD/STZ diabetic mouse model, the expression levels of pyroptosis-related proteins, including NLRP3 inflammasome, caspase-1, and GSDMD, were increased (130). Chen J et al. also found the same result, NLRP3 inflammasome recognizes risk signals, activates GSDMD, and inflammatory substances such as caspase-1 and IL-1β trigger pyroptosis and induce an inflammatory response. And it was confirmed by the TUNEL experiment that pyroptosis promotes the progress of DN (131). In addition, other studies have demonstrated that pyroptosis mediated by the NLRP3 inflammasome is associated with inflammation and fibrosis in DN, leading to aggravation of DN, the phenomenon was improved after the use of the caspase-1 inhibitor VX-765 (132–134). Notably, in renal tubular epithelial cells (TECs) and podocytes, NLRP3 inflammasome-mediated pyroptosis is critical in promoting DN progression (135–137).
Podocyte pyroptosis
Podocytes are highly differentiated atypical epithelial cells of the kidney that are non-dividing, unique insulin-sensitive cells in the glomerulus. Participate in forming the filtration barrier and maintain the normal filtration function of the glomerulus (138). Podocyte loss is a central factor in DN proteinuria (139). Many studies have found podocyte pyroptosis closely related to diabetic nephropathy (140). HG inhibited the survival of podocytes in a dose-dependent manner and increased the levels of pyroptosis-related proteins such as ROS, IL-1β, and IL-18 in cells (141). Notably, activation of the NLRP3 inflammasome in podocytes promotes glomerular inflammation and glomerulosclerosis progression (142). In addition, D-ribose promotes NLRP3 inflammasome activation in type 2 diabetes to induce podocyte injury and glomerulosclerosis, the caspase-1 inhibitor YvAD significantly blocks podocyte injury (143). ABAIS et al. demonstrated that in a diabetes model, the protein expression levels of caspase-11 and GSDMD-N in podocytes were increased, and the expression of podocyte markers nephrin and podocin were decreased, podocyte loss and foot process fusion, promoting the expression of inflammatory factors such as NF-κB, IL-1β and IL-18 (140). Recent studies have found that the mammalian target of rapamycin (mTOR) regulates inflammation by binding to NLRP3 (144–146). Wang T et al. show that mTOR and NF-κB inhibitors reduce renal podocyte injury (147). In addition to the mTOR/NLRP3 signaling pathway, miRNAs can mediate the activation of the NLRP3 inflammasome and trigger podocyte pyroptosis under high glucose conditions. For example, Ding et al. demonstrated that miR-21-5p induces podocyte pyroptosis (148). In the study of Zhan et al., Long Non-Coding RNA (lncRNA) NEAT1 promoted podocyte pyroptosis by regulating miR-34c and regulated the expression of NLRP3, Caspase-1, and IL-1β in a mouse model of diabetes (129). Thioredoxin-interacting protein (TXNIP)/reactive oxygen species (ROS)/NLRP3 pathway is also involved in mediating podocyte pyroptosis and promoting the progression of DN (135). These findings above highlight the role of podocyte pyroptosis induced by the NLRP3/Caspase-1/IL-1β axis in diabetic nephropathy. Some of the latest cell studies also inhibit podocyte pyroptosis by interfering NLRP3 inflammasome signaling pathway, such as using the NLRP3 inflammasome inhibitor MCC950, which is expected to be used in treating diabetic nephropathy (135, 149–151).
Tubular epithelial cell pyroptosis
TECs are responsible for renal reabsorption and are closely related to the deterioration of renal function. The damage of TECs is also a critical link in DKD (152). Under HG conditions, TECs are more susceptible to glucose and lipid metabolism disorders, inflammatory responses, and hemodynamic changes, and the high-glucose environment produces ROS and releases various inflammatory factors, resulting in renal interstitial inflammation and fibrosis (122). Zhang et al. found that Caspase-11-mediated pyroptosis of TECs plays an essential role in acute kidney injury (137). In addition, lncRNAs are closely related to the progression of DN; for example, Xie et al. found that lncRNA GAS5 regulates the NLRP3 inflammasome-caspase-1 axis to mediate the pyroptosis of TECs (153). Similarly, Zhu et al. confirmed that the expression of NLRP3, caspase-1, IL-1β, p-IL-1β and GSDMD-N was up-regulated in HG-induced human tubular cells (HK-2), and the pyroptosis of HK-2 is associated with lncRNA KCNQ1OT1 (154). In the study of Wang et al., HG activated TLR4/NF-κB signaling pathway to mediate the pyroptosis of GSDMD-related TECs, and TLR4 inhibitor TAK-242 significantly ameliorated the damage of TECs (155). Interestingly, TXINP/NLRP3 pathway is also involved in mediating TECs pyroptosis; Ke et al. demonstrated that HG activates TXINP/NLRP3 pathway to mediate NRK-52E cell pyroptosis and kidney injury (134). HG also promotes TXNIP/NLRP3/caspase-1 pathway activation to mediate HK-2 pyroptosis (156).
Diabetic retinopathy
As a common microvascular complication in diabetic patients, diabetic retinopathy (DR) is one of the leading causes of visual impairment in adults worldwide. Activation of the NLRP3 inflammasome may be involved in the pathogenesis of DR (157). For example, in the study by Yu et al., caspase-1 and GSDMD were activated after induction using advanced glycation end-products-modified bovine serum albumin (AGE-BSA), promoting inflammatory factors IL-1β, IL-18, and LDH release (158). Loukovaara et al. found elevated levels of pyroptosis-related proteins in DR (159). After induction with STZ, the expression levels of NF-κB, NLRP3, and caspase-1 were up-regulated in DR (160). In addition, HG can induce pyroptosis of human retinal microvascular endothelial cells (HRMECs) and human retinal pericytes (HRPs) (161, 162). NLRP3 inflammasome promotes cone cell death in a P23H rhodopsin retinal degeneration model (163). Za et al. also demonstrated HG-induced retinal pigment epithelium (RPE) cell pyroptosis (164). In recent years, more and more studies have been conducted on the mechanism of DR pyroptosis. RNA, methyltransferase-like protein 3 (METTL3), P2X7 purinergic receptor (P2X7R)/NLRP3, and ROS/TXNIP/NLRP3 pathways may all be related to DR pyroptosis (158, 164, 165). Targeted intervention in the activation of NLRP3 inflammasome may be beneficial for the prevention and treatment of DR.
Macrovascular and Cardiac Complications
Atherosclerosis
The risk of macrovascular disease is significantly increased in diabetes. Atherosclerosis (AS) in the aorta, coronary artery, and cerebral basilar artery is one of the complications of diabetes and the leading cause of cardiovascular events in patients with diabetes (166). A growing number of studies have demonstrated that many risk factors for type 2 diabetes, such as high glucose and high fat, can activate the NLRP3 inflammasome in endothelial cells (ECs) and macrophages to mediate pyroptosis and exacerbate the progression of AS (167). NLRP3 inflammasome and pyroptosis in atherosclerotic plaques are positively correlated with plaque rupture and vascular inflammation (168). In the study by An et al., carotid artery injury in T2D model rats was associated with elevated levels of NLRP3, caspase-1, and IL-1β (169). Rat aortic AS was associated with tissue activation of the NLRP3 inflammasome and NF-κB signaling in the Zucker diabetic fat (ZDF) model rat (170). Song et al. confirmed that HG promotes NLRP3 inflammasome activation and IL-1β secretion in ECs (171). Furthermore, in the study of Chen et al., HG increased the expression levels of NLRP3, caspase-1, and IL-1β in human arterial smooth muscle cells (HASMCs) (172). NLRP3 inflammasome, caspase-1, IL-1β, and IL-18 expression were upregulated in aortic ECs and vascular smooth muscle cells (VSMCs) in HFD-fed mice (173). The above studies further confirmed that pyroptosis mediated by the NLRP3 inflammasome promotes the progression of AS in a diabetes model, and targeting the pyroptosis associated with the NLRP3 inflammasome may have a potential therapeutic effect on type 2 diabetes with AS.
Diabetic cardiomyopathy
DCM is a prevalent CVD, characterized by systolic dysfunction and left ventricular hypertrophy. Many risk factors, such as hyperglycemia, insulin resistance, increased oxidative stress, mitochondrial dysfunction, cardiomyocyte damage, and endothelial dysfunction, contribute to the development of DCM (174). A previous study found that the hearts overexpressed NLRP3, caspase-1, and IL-1 in diabetic rats (175). Pyroptosis associated with the NLRP3 inflammasome plays an important role in the pathogenesis of DCM (176). Silencing the NLRP3 gene improves cardiac inflammation, pyroptosis, and myocardial function (177). In cardiac tissue, pyroptosis occurs mainly in cardiomyocytes (CMs) and cardiac fibroblasts (CFs) (178–180). The death of CMs and CFs leads to cardiac remodeling and left ventricular dysfunction.
Cardiomyocyte pyroptosis in DCM
Cardiomyocytes maintain the systolic and diastolic functions of the heart and ensure the blood supply of the whole body (123). Under high glucose conditions, the NLRP3 inflammasome was activated through multiple pathways to induce pyroptosis in CMs. For example, hyperglycemia promoted cardiomyocyte pyroptosis by activating the AMPK-TXNIP/NLRP3 signaling pathway (134, 181), and pyroptosis-associated TLR4 and NLRP3 inflammasome expression was increased in HG-treated H9C2 cardiomyocytes (182). HG also induced cardiomyocyte H9c2 pyroptosis by activating the NF-kB/NLRP3 pathway (177). ROS/NLRP3 and JNK/NLRP3 signaling pathways also promoted cardiomyocyte pyroptosis (183, 184). In addition, ELAV-like protein 1 (ELAVL1), LncRNA Kcnq1ot1, and miRNA were all involved in regulating cardiomyocyte pyroptosis in DCM (185–187). The death of CMs promotes DCM progression, leading to ventricular remodeling and even heart failure.
Cardiac fibroblasts Pyroptosis in DCM
Cardiac fibrosis is one of the main pathological features of DCM, and CFs injury plays an essential role in this process. CFs are considered semi-occupational inflammatory cells that play an immunomodulatory role in the heart. Pyroptosis mediated by the NLRP3 inflammasome as pro-inflammatory programmed cell death is closely related to the damage of CFs (188). The NLRP3 inflammasome was activated with the participation of LncRNA, ROS, miRNA, and other mediators in the HG state, leading to cardiac fibroblast pyroptosis, promoting collagen synthesis and aggravating cardiac tissue fibrosis (189, 190). Some recent studies have further confirmed that CFs pyroptosis plays an important role in the pathogenesis of DCM (191, 192). It is necessary to explore further the mechanism of CFs pyroptosis for the prevention and treatment of DCM.
Diabetic neuropathy
Extensive research evidence suggests that pyroptosis is also an important regulatory mechanism for diabetic neuropathy (125). Pyroptosis can lead to neuronal death and exacerbates diabetic neuropathy processes such as ischemic stroke, cognitive impairment, spinal cord injury (SCI), and peripheral neuropathy (193). In addition, pyroptosis mediated by the NLRP3 inflammasome also occurs in the optic and enteric nerves under high glucose conditions (194, 195).
Diabetic encephalopathy
In recent years, pyroptosis in diabetic encephalopathy has received more and more attention. T2D induces pyroptosis by inducing activation of the NLRP3 inflammasome, aggravating neurodegeneration, cerebral infarction, and progressive cognitive decline (125). For example, Hong et al. found that the NLRP3 inhibitor MCC950 ameliorated cerebral ischemia/reperfusion (I/R) injury and reduced ischemic stroke risk in diabetic mice (196). In Wang et al., microglia pyroptosis exacerbates I/R injury, and NLRP3-specific inhibitor MCC950 ameliorated cerebral I/R injury in diabetic mice (197); Li et al. also found that hippocampal neurons in STZ-induced diabetes model mice mediated pyroptosis through the NLRP3 signaling pathway, accompanied by mouse depression-like behaviors (198). Similarly, Che et al. found that neuronal expression levels of NLRP3 inflammasome and pyroptosis were increased (199). Pyroptosis mediated by the NLRP3 inflammasome is a complex process in the progression of DE, and both lncRNAs and miRNAs play important roles in the process of pyroptosis (197, 199). Further exploring the mechanism of pyroptosis, especially the NLRP3 inflammasome signaling pathway, may be an essential strategy for the therapy of DE.
Diabetic peripheral neuropathy
As one of the common complications of diabetes, diabetic peripheral neuropathy (DPN) is characterized by chronic inflammation, axonal degeneration, loss of unmyelinated fibers, and irreversible neuronal damage (165). Excessive ROS production leading to NLRP3 inflammasome activation promotes the level of pyroptosis in DPN (200). In Cheng et al.’s study, HG-induced the activation of NF-κB and NLRP3 inflammasome mediated pyroptosis in Schwann cells and promoted DPN progression (201). Similarly, Li et al. demonstrated that high glucose exacerbates demyelination in a mouse model of T2DM (202). Furthermore, in the study by Xu et al., high fat promoted the activation of the TXNIP/NLRP3 inflammasome in a diabetic mouse model (203). At the same time, miRNAs were also involved in the process of neuronal pyroptosis in diabetic mice (199). These findings proved that pyroptosis related to NLRP3 plays an important role in the development of DPN.
Other complications
Pyroptosis mediated by the NLRP3 inflammasome also promoted the progression of diabetes-associated nonalcoholic fatty liver disease (NAFLD) syndrome. HG and saturated fatty acids acted together in the liver to exacerbate inflammation and endoplasmic reticulum stress to destabilize mitochondria (204), and long-term inflammatory response led to hepatocyte pyroptosis and promoted liver fibrosis (205). Activation of inflammasome directly stimulated hepatic stellate cells (HSCs) to increase the secretion of matrix metalloproteinases (MMPs) and accelerate the process of liver fibrosis (206). In addition, pyroptosis of stellate cells mediated by NLRP3 inflammasome further promotes liver fibrosis (207). After a specific knockout of the NLRP3 gene in hepatocytes, liver inflammation was significantly reduced (208). These studies suggested that pyroptosis mediated by NLRP3 inflammasome is vital for preventing and treating NAFLD.
Potential drugs and agents that inhibit NLRP3 inflammasome signaling pathways
In recent years, there have been more and more studies on the mechanism of T2D pyroptosis, and many clinical drugs and compounds have demonstrated specific anti-pyroptosis effects (Table 1). For example, metformin, a first-line drug for diabetes treatment, inhibited the activation of NLRP3 inflammasome and cardiomyocyte pyroptosis (209–211), reducing myocardial ischemia-reperfusion injury (210). GLP-1 receptor agonists liraglutide and exenatide reduced neurological damage and inhibited NAFLD and cardiomyocyte pyroptosis in diabetic rats by inhibiting NLRP3 inflammasome activation (212–215); Similarly, exendin-4 also inhibited the activation of NLRP3 under HG conditions and alleviated cardiomyocyte pyroptosis (181). Furthermore, SGLT-2 inhibitor dapagliflozin and DPP4 inhibitor saxagliptin reduced NLRP3 inflammasome activation and delayed the progression of DCM in diabetic mice (216).
Various active substances in traditional Chinese medicine also improved the pyroptosis of diabetes and its complications by inhibiting the NLRP3 inflammasome pathway, such as ginsenoside Rg5 reduced kidney damage by inhibiting the activation of NF-κB/NLRP3 signaling pathway in the HT/STZ diabetic mouse model (217). Huangkui capsule attenuated tubular epithelial-mesenchymal transition in diabetic nephropathy mice by inhibiting TLR4/NF-κB/NLRP3 pathway (218). Gypenosides alleviated diabetic myocardial injury by inhibiting ROS/NLRP3 inflammasome activation (184). Notably, Salidroside alleviated NAFLD in HT diet mice by regulating TXNIP/NLRP3 pathway (219). Sulforaphane inhibited the activation of NLRP3 inflammasome against diabetic retinopathy (220). In addition, Jinmaitong also regulated the NLRP3 pathway to improve STZ-induced DPN in rats (165). In the study of Bai et al., a detailed summary of traditional Chinese medicines targeting the NLRP3 inflammasome pathway to interfere with diabetes progression (167).
Some natural substances also delayed the progression of diabetes and its complications by inhibiting the NLRP3 inflammasome-mediated pyroptosis pathway. For example, melatonin alleviated the pyroptosis of endothelial cells, slowed the progression of atherosclerosis, and inhibited the pyroptosis of neurons and cardiomyocytes in a diabetic mouse model by regulating the NLRP3 axis (199, 221, 222). Similarly, H3 relaxin attenuated pyroptosis in DR and inhibited fibrosis and inflammation in cardiomyocytes of diabetic rats (223, 224). In addition, sodium butyrate (Nab) reduced the pyroptosis of glomerular endothelial cells under HG conditions, and miRNA and LncRNA also played an essential role in the pyroptosis of diabetes and its complications. Targeted knockout or inhibition of RNA expression can reduce the activation of NLRP3 inflammation and delay the progression of diabetes (42, 225–227). According to the existing research evidence, pyroptosis mediated by the NLRP3 inflammasome was commonly found in T2D and its complications. The use of drugs or NLRP3 inflammasome inhibitor MCC950 (196), or even knockout of the NLRP3 gene to inhibit pyroptosis seem to have some effect on T2D Treatment (Figure 3) (177). Therefore, targeting the NLRP3 inflammasome may lead to more options for the treatment of diabetic patients in the future.
Summary and outlook
As a complex metabolic disease, the pathogenesis and complications of diabetes continue to progress. Therefore, the exploration of new treatment modalities and intervention mechanisms is necessary. As a new form of programmed cell death, pyroptosis plays a vital role in the occurrence and development of many diseases. Numerous studies have shown that intervening in the NLRP3 inflammasome signaling pathway can attenuate pyroptosis under HG conditions and delay the progression of complications in animal models of T2D. Targeted regulation of pyroptosis mediated by the NLRP3 inflammasome appears to play a critical role in the progression of diabetes. However, there are still some problems that need to be solved. For example, current studies are all animal models or cell experiments, which are not enough to support targeting the NLRP3 inflammasome to prevent and treat T2D; in addition, the proportion of pyroptosis mediated by the NLRP3 inflammasome in the progression of diabetes is yet unknown, and the research on intervening the mechanism of pyroptosis to delay the progression of T2D is still challenging. However, it is undeniable that the targeted regulation of NLRP3 inflammasome activation has some effect in the treatment of T2D, and some drugs have gradually shown anti-pyroptotic effects. Therefore, an in-depth study of the mechanism of pyroptosis mediated by NLRP3 inflammasome and potential anti-pyroptotic agents may bring some new strategies for treating T2D and drug development.
Author contributions
XL wrote the manuscript. G-YX and Y-JS participated in literature collection and producing the figures. TG participated in literature collection and edited the manuscript. Q-ML edited the manuscript. XL and Q-ML conceived the study. All authors contributed to the article and approved the submitted version.
Conflict of interest
The authors declare that the research was conducted in the absence of any commercial or financial relationships that could be construed as a potential conflict of interest.
Publisher’s note
All claims expressed in this article are solely those of the authors and do not necessarily represent those of their affiliated organizations, or those of the publisher, the editors and the reviewers. Any product that may be evaluated in this article, or claim that may be made by its manufacturer, is not guaranteed or endorsed by the publisher.
References
1. Sladek R. The many faces of diabetes: addressing heterogeneity of a complex disease. Lancet Diabetes Endocrinol (2018) 6:348–9. doi: 10.1016/S2213-8587(18)30070-6
2. Roden M, Shulman GI. The integrative biology of type 2 diabetes. Nature (2019) 576:51–60. doi: 10.1038/s41586-019-1797-8
3. Prattichizzo F, De Nigris V, Spiga R, Mancuso E, La Sala L, Antonicelli R, et al. Inflammageing and metaflammation: The yin and yang of type 2 diabetes. Ageing Res Rev (2018) 41:1–17. doi: 10.1016/j.arr.2017.10.003
4. Prattichizzo F, De Nigris V, Micheloni S, La Sala L, Ceriello A. Increases in circulating levels of ketone bodies and cardiovascular protection with SGLT2 inhibitors: Is low-grade inflammation the neglected component? Diabetes Obes Metab (2018) 20:2515–22. doi: 10.1111/dom.13488
5. Hotamisligil GS. Inflammation, metaflammation and immunometabolic disorders. Nature (2017) 542:177–85. doi: 10.1038/nature21363
6. Rawshani A, Rawshani A, Franzén S, Eliasson B, Svensson AM, Miftaraj M, et al. Mortality and cardiovascular disease in type 1 and type 2 diabetes. N Engl J Med (2017) 376:1407–18. doi: 10.1056/NEJMoa1608664
7. Kahn SE, Cooper ME, Del Prato S. Pathophysiology and treatment of type 2 diabetes: perspectives on the past, present, and future. Lancet (2014) 383:1068–83. doi: 10.1016/S0140-6736(13)62154-6
8. Nauck MA, Wefers J, Meier JJ. Treatment of type 2 diabetes: challenges, hopes, and anticipated successes. Lancet Diabetes Endocrinol (2021) 9:525–44. doi: 10.1016/S2213-8587(21)00113-3
9. Perreault L, Skyler JS, Rosenstock J. Novel therapies with precision mechanisms for type 2 diabetes mellitus. Nat Rev Endocrinol (2021) 17:364–77. doi: 10.1038/s41574-021-00489-y
10. Demir S, Nawroth PP, Herzig S, Ekim Üstünel B. Emerging targets in type 2 diabetes and diabetic complications. Adv Sci (Weinh) (2021) 8:e2100275. doi: 10.1002/advs.202100275
11. Wentworth JM, Fourlanos S, Harrison LC. Reappraising the stereotypes of diabetes in the modern diabetogenic environment. Nat Rev Endocrinol (2009) 5:483–9. doi: 10.1038/nrendo.2009.149
12. Donath MY, Shoelson SE. Type 2 diabetes as an inflammatory disease. Nat Rev Immunol (2011) 11:98–107. doi: 10.1038/nri2925
13. Kamradt T, Mitchison NA. Tolerance and autoimmunity. N Engl J Med (2001) 344:655–64. doi: 10.1056/NEJM200103013440907
14. Christgen S, Tweedell RE, Kanneganti TD. Programming inflammatory cell death for therapy. Pharmacol Ther (2022) 232:108010. doi: 10.1016/j.pharmthera.2021.108010
15. Friedlander AM. Macrophages are sensitive to anthrax lethal toxin through an acid-dependent process. J Biol Chem (1986) 261:7123–6. doi: 10.1016/S0021-9258(17)38364-3
16. D'Souza CA, Heitman J. Dismantling the cryptococcus coat. Trends Microbiol (2001) 9:112–3. doi: 10.1016/S0966-842X(00)01945-4
17. Shi J, Zhao Y, Wang K, Shi X, Wang Y, Huang H, et al. Cleavage of GSDMD by inflammatory caspases determines pyroptotic cell death. Nature (2015) 526:660–5. doi: 10.1038/nature15514
18. Ding J, Wang K, Liu W, She Y, Sun Q, Shi J, et al. Pore-forming activity and structural autoinhibition of the gasdermin family. Nature (2016) 535:111–6. doi: 10.1038/nature18590
19. Liu X, Zhang Z, Ruan J, Pan Y, Magupalli VG, Wu H, et al. Inflammasome-activated gasdermin d causes pyroptosis by forming membrane pores. Nature (2016) 535:153–8. doi: 10.1038/nature18629
20. Aglietti RA, Dueber EC. Recent insights into the molecular mechanisms underlying pyroptosis and gasdermin family functions. Trends Immunol (2017) 38:261–71. doi: 10.1016/j.it.2017.01.003
21. Kesavardhana S, Malireddi RKS, Kanneganti TD. Caspases in cell death, inflammation, and pyroptosis. Annu Rev Immunol (2020) 38:567–95. doi: 10.1146/annurev-immunol-073119-095439
22. Brennan MA, Cookson BT. Salmonella induces macrophage death by caspase-1-dependent necrosis. Mol Microbiol (2000) 38:31–40. doi: 10.1046/j.1365-2958.2000.02103.x
23. Boise LH, Collins CM. Salmonella-induced cell death: apoptosis, necrosis or programmed cell death? Trends Microbiol (2001) 9:64–7. doi: 10.1016/S0966-842X(00)01937-5
24. Elmore S. Apoptosis: a review of programmed cell death. Toxicol Pathol (2007) 35:495–516. doi: 10.1080/01926230701320337
25. Kayagaki N, Warming S, Lamkanfi M, Vande Walle L, Louie S, Dong J, et al. Non-canonical inflammasome activation targets caspase-11. Nature (2011) 479:117–21. doi: 10.1038/nature10558
26. Kolb R, Liu GH, Janowski AM, Sutterwala FS, Zhang W. Inflammasomes in cancer: a double-edged sword. Protein Cell (2014) 5:12–20. doi: 10.1007/s13238-013-0001-4
27. Wang Y, Gao W, Shi X, Ding J, Liu W, He H, et al. Chemotherapy drugs induce pyroptosis through caspase-3 cleavage of a gasdermin. Nature (2017) 547:99–103. doi: 10.1038/nature22393
28. Orning P, Weng D, Starheim K, Ratner D, Best Z, Lee B, et al. Pathogen blockade of TAK1 triggers caspase-8-dependent cleavage of gasdermin d and cell death. Science (2018) 362:1064–9. doi: 10.1126/science.aau2818
29. Sarhan J, Liu BC, Muendlein HI, Li P, Nilson R, Tang AY, et al. Caspase-8 induces cleavage of gasdermin d to elicit pyroptosis during yersinia infection. Proc Natl Acad Sci U.S.A. (2018) 115:E10888–e10897. doi: 10.1073/pnas.1809548115
30. Kayagaki N, Stowe IB, Lee BL, O'Rourke K, Anderson K, Warming S, et al. Caspase-11 cleaves gasdermin d for non-canonical inflammasome signalling. Nature (2015) 526:666–71. doi: 10.1038/nature15541
31. Yu P, Zhang X, Liu N, Tang L, Peng C, Chen X. Pyroptosis: mechanisms and diseases. Signal Transduct Target Ther (2021) 6:128. doi: 10.1038/s41392-021-00507-5
32. de Vasconcelos NM, Van Opdenbosch N, Van Gorp H, Martín-Pérez R, Zecchin A, Vandenabeele P, et al. An apoptotic caspase network safeguards cell death induction in pyroptotic macrophages. Cell Rep (2020) 32:107959. doi: 10.1016/j.celrep.2020.107959
33. Aziz M, Jacob A, Wang P. Revisiting caspases in sepsis. Cell Death Dis (2014) 5:e1526. doi: 10.1038/cddis.2014.488
34. Vanden Berghe T, Demon D, Bogaert P, Vandendriessche B, Goethals A, Depuydt B, et al. Simultaneous targeting of IL-1 and IL-18 is required for protection against inflammatory and septic shock. Am J Respir Crit Care Med (2014) 189:282–91. doi: 10.1164/rccm.201308-1535OC
35. Emran AA, Tseng HY, Coleman MC, Tiffen J, Cook S, McGuire HM, et al. Do innate killing mechanisms activated by inflammasomes have a role in treating melanoma? Pigment Cell Melanoma Res (2020) 33:660–70. doi: 10.1111/pcmr.12870
36. Fang Y, Tian S, Pan Y, Li W, Wang Q, Tang Y, et al. Pyroptosis: A new frontier in cancer. BioMed Pharmacother (2020) 121:109595. doi: 10.1016/j.biopha.2019.109595
37. Komada T, Muruve DA. The role of inflammasomes in kidney disease. Nat Rev Nephrol (2019) 15:501–20. doi: 10.1038/s41581-019-0158-z
38. Rogers C, Fernandes-Alnemri T, Mayes L, Alnemri D, Cingolani G, Alnemri ES. Cleavage of DFNA5 by caspase-3 during apoptosis mediates progression to secondary necrotic/pyroptotic cell death. Nat Commun (2017) 8:14128. doi: 10.1038/ncomms14128
39. Hou J, Zhao R, Xia W, Chang CW, You Y, Hsu JM, et al. PD-L1-mediated gasdermin c expression switches apoptosis to pyroptosis in cancer cells and facilitates tumour necrosis. Nat Cell Biol (2020) 22:1264–75. doi: 10.1038/s41556-020-0575-z
40. Zheng M, Williams EP, Malireddi RKS, Karki R, Banoth B, Burton A, et al. Impaired NLRP3 inflammasome activation/pyroptosis leads to robust inflammatory cell death via caspase-8/RIPK3 during coronavirus infection. J Biol Chem (2020) 295:14040–52. doi: 10.1074/jbc.RA120.015036
41. Zhou B, Zhang JY, Liu XS, Chen HZ, Ai YL, Cheng K, et al. Tom20 senses iron-activated ROS signaling to promote melanoma cell pyroptosis. Cell Res (2018) 28:1171–85. doi: 10.1038/s41422-018-0090-y
42. Mamun AA, Wu Y, Nasrin F, Akter A, Taniya MA, Munir F, et al. Role of pyroptosis in diabetes and its therapeutic implications. J Inflamm Res (2021) 14:2187–206. doi: 10.2147/JIR.S291453
43. Christgen S, Kanneganti TD. Inflammasomes and the fine line between defense and disease. Curr Opin Immunol (2020) 62:39–44. doi: 10.1016/j.coi.2019.11.007
44. Frank D, Vince JE. Pyroptosis versus necroptosis: similarities, differences, and crosstalk. Cell Death Differ (2019) 26:99–114. doi: 10.1038/s41418-018-0212-6
45. Jackson DN, Theiss AL. Gut bacteria signaling to mitochondria in intestinal inflammation and cancer. Gut Microbes (2020) 11:285–304. doi: 10.1080/19490976.2019.1592421
46. Christgen S, Place DE, Kanneganti TD. Toward targeting inflammasomes: insights into their regulation and activation. Cell Res (2020) 30:315–27. doi: 10.1038/s41422-020-0295-8
47. Man SM, Kanneganti TD. Regulation of inflammasome activation. Immunol Rev (2015) 265:6–21. doi: 10.1111/imr.12296
48. Man SM, Karki R, Kanneganti TD. Molecular mechanisms and functions of pyroptosis, inflammatory caspases and inflammasomes in infectious diseases. Immunol Rev (2017) 277:61–75. doi: 10.1111/imr.12534
49. Zitvogel L, Kepp O, Galluzzi L, Kroemer G. Inflammasomes in carcinogenesis and anticancer immune responses. Nat Immunol (2012) 13:343–51. doi: 10.1038/ni.2224
50. Kawai T, Akira S. Toll-like receptors and their crosstalk with other innate receptors in infection and immunity. Immunity (2011) 34:637–50. doi: 10.1016/j.immuni.2011.05.006
51. Barton GM, Medzhitov R. Toll-like receptor signaling pathways. Science (2003) 300:1524–5. doi: 10.1126/science.1085536
52. Lamkanfi M, Dixit VM. Inflammasomes and their roles in health and disease. Annu Rev Cell Dev Biol (2012) 28:137–61. doi: 10.1146/annurev-cellbio-101011-155745
53. Broz P, Dixit VM. Inflammasomes: mechanism of assembly, regulation and signalling. Nat Rev Immunol (2016) 16:407–20. doi: 10.1038/nri.2016.58
54. Lamkanfi M, Dixit VM. Mechanisms and functions of inflammasomes. Cell (2014) 157:1013–22. doi: 10.1016/j.cell.2014.04.007
55. Ewald SE, Chavarria-Smith J, Boothroyd JC. NLRP1 is an inflammasome sensor for toxoplasma gondii. Infect Immun (2014) 82:460–8. doi: 10.1128/IAI.01170-13
56. Huang M, Zhang X, Toh GA, Gong Q, Wang J, Han Z, et al. Structural and biochemical mechanisms of NLRP1 inhibition by DPP9. Nature (2021) 592:773–7. doi: 10.1038/s41586-021-03320-w
57. Hornung V, Ablasser A, Charrel-Dennis M, Bauernfeind F, Horvath G, Caffrey DR, et al. AIM2 recognizes cytosolic dsDNA and forms a caspase-1-activating inflammasome with ASC. Nature (2009) 458:514–8. doi: 10.1038/nature07725
58. Roberts TL, Idris A, Dunn JA, Kelly GM, Burnton CM, Hodgson S, et al. HIN-200 proteins regulate caspase activation in response to foreign cytoplasmic DNA. Science (2009) 323:1057–60. doi: 10.1126/science.1169841
59. Jin T, Perry A, Jiang J, Smith P, Curry JA, Unterholzner L, et al. Structures of the HIN domain:DNA complexes reveal ligand binding and activation mechanisms of the AIM2 inflammasome and IFI16 receptor. Immunity (2012) 36:561–71. doi: 10.1016/j.immuni.2012.02.014
60. Matyszewski M, Morrone SR, Sohn J. Digital signaling network drives the assembly of the AIM2-ASC inflammasome. Proc Natl Acad Sci U.S.A. (2018) 115:E1963–e1972. doi: 10.1073/pnas.1712860115
61. Xu H, Yang J, Gao W, Li L, Li P, Zhang L, et al. Innate immune sensing of bacterial modifications of rho GTPases by the pyrin inflammasome. Nature (2014) 513:237–41. doi: 10.1038/nature13449
62. Kim ML, Chae JJ, Park YH, De Nardo D, Stirzaker RA, Ko HJ, et al. Aberrant actin depolymerization triggers the pyrin inflammasome and autoinflammatory disease that is dependent on IL-18, not IL-1β. J Exp Med (2015) 212:927–38. doi: 10.1084/jem.20142384
63. Okondo MC, Johnson DC, Sridharan R, Go EB, Chui AJ, Wang MS, et al. DPP8 and DPP9 inhibition induces pro-caspase-1-dependent monocyte and macrophage pyroptosis. Nat Chem Biol (2017) 13:46–53. doi: 10.1038/nchembio.2229
64. Chavarría-Smith J, Vance RE. Direct proteolytic cleavage of NLRP1B is necessary and sufficient for inflammasome activation by anthrax lethal factor. PloS Pathog (2013) 9:e1003452. doi: 10.1371/journal.ppat.1003452
65. Levinsohn JL, Newman ZL, Hellmich KA, Fattah R, Getz MA, Liu S, et al. Anthrax lethal factor cleavage of Nlrp1 is required for activation of the inflammasome. PloS Pathog (2012) 8:e1002638. doi: 10.1371/journal.ppat.1002638
66. Zindel J, Kubes P. DAMPs, PAMPs, and LAMPs in immunity and sterile inflammation. Annu Rev Pathol (2020) 15:493–518. doi: 10.1146/annurev-pathmechdis-012419-032847
67. Swanson KV, Deng M, Ting JP. The NLRP3 inflammasome: molecular activation and regulation to therapeutics. Nat Rev Immunol (2019) 19:477–89. doi: 10.1038/s41577-019-0165-0
68. Greaney AJ, Leppla SH, Moayeri M. Bacterial exotoxins and the inflammasome. Front Immunol (2015) 6:570. doi: 10.3389/fimmu.2015.00570
69. Mathur A, Feng S, Hayward JA, Ngo C, Fox D, Atmosukarto II, et al. A multicomponent toxin from bacillus cereus incites inflammation and shapes host outcome via the NLRP3 inflammasome. Nat Microbiol (2019) 4:362–74. doi: 10.1038/s41564-018-0318-0
70. Mariathasan S, Weiss DS, Newton K, McBride J, O'Rourke K, Roose-Girma M, et al. Cryopyrin activates the inflammasome in response to toxins and ATP. Nature (2006) 440:228–32. doi: 10.1038/nature04515
71. He Y, Zeng MY, Yang D, Motro B, Núñez G. NEK7 is an essential mediator of NLRP3 activation downstream of potassium efflux. Nature (2016) 530:354–7. doi: 10.1038/nature16959
72. Shi H, Wang Y, Li X, Zhan X, Tang M, Fina M, et al. NLRP3 activation and mitosis are mutually exclusive events coordinated by NEK7, a new inflammasome component. Nat Immunol (2016) 17:250–8. doi: 10.1038/ni.3333
73. Schmid-Burgk JL, Chauhan D, Schmidt T, Ebert TS, Reinhardt J, Endl E, et al. (Clustered regularly interspaced short palindromic repeats) screen identifies NEK7 as an essential component of NLRP3 inflammasome activation. J Biol Chem (2016) 291:103–9. doi: 10.1074/jbc.C115.700492
74. Kofoed EM, Vance RE. Innate immune recognition of bacterial ligands by NAIPs determines inflammasome specificity. Nature (2011) 477:592–5. doi: 10.1038/nature10394
75. Zhao Y, Yang J, Shi J, Gong YN, Lu Q, Xu H, et al. The NLRC4 inflammasome receptors for bacterial flagellin and type III secretion apparatus. Nature (2011) 477:596–600. doi: 10.1038/nature10510
76. Duncan JA, Canna SW. The NLRC4 inflammasome. Immunol Rev (2018) 281:115–23. doi: 10.1111/imr.12607
77. Fernandes-Alnemri T, Yu JW, Juliana C, Solorzano L, Kang S, Wu J, et al. The AIM2 inflammasome is critical for innate immunity to francisella tularensis. Nat Immunol (2010) 11:385–93. doi: 10.1038/ni.1859
78. Aubert DF, Xu H, Yang J, Shi X, Gao W, Li L, et al. A burkholderia type VI effector deamidates rho GTPases to activate the pyrin inflammasome and trigger inflammation. Cell Host Microbe (2016) 19:664–74. doi: 10.1016/j.chom.2016.04.004
79. Van Gorp H, Saavedra PH, de Vasconcelos NM, Van Opdenbosch N, Vande Walle L, Matusiak M, et al. Familial Mediterranean fever mutations lift the obligatory requirement for microtubules in pyrin inflammasome activation. Proc Natl Acad Sci U.S.A. (2016) 113:14384–9. doi: 10.1073/pnas.1613156113
80. Ratner D, Orning MP, Proulx MK, Wang D, Gavrilin MA, Wewers MD, et al. The yersinia pestis effector YopM inhibits pyrin inflammasome activation. PloS Pathog (2016) 12:e1006035. doi: 10.1371/journal.ppat.1006035
81. Chung LK, Park YH, Zheng Y, Brodsky IE, Hearing P, Kastner DL, et al. The yersinia virulence factor YopM hijacks host kinases to inhibit type III effector-triggered activation of the pyrin inflammasome. Cell Host Microbe (2016) 20:296–306. doi: 10.1016/j.chom.2016.07.018
82. Sollberger G, Strittmatter GE, Garstkiewicz M, Sand J, Beer HD. Caspase-1: the inflammasome and beyond. Innate Immun (2014) 20:115–25. doi: 10.1177/1753425913484374
83. Chen X, He WT, Hu L, Li J, Fang Y, Wang X, et al. Pyroptosis is driven by non-selective gasdermin-d pore and its morphology is different from MLKL channel-mediated necroptosis. Cell Res (2016) 26:1007–20. doi: 10.1038/cr.2016.100
84. Sborgi L, Rühl S, Mulvihill E, Pipercevic J, Heilig R, Stahlberg H, et al. GSDMD membrane pore formation constitutes the mechanism of pyroptotic cell death. EMBO J (2016) 35:1766–78. doi: 10.15252/embj.201694696
85. Bergsbaken T, Fink SL, Cookson BT. Pyroptosis: host cell death and inflammation. Nat Rev Microbiol (2009) 7:99–109. doi: 10.1038/nrmicro2070
86. He WT, Wan H, Hu L, Chen P, Wang X, Huang Z, et al. Gasdermin d is an executor of pyroptosis and required for interleukin-1β secretion. Cell Res (2015) 25:1285–98. doi: 10.1038/cr.2015.139
87. Hagar JA, Powell DA, Aachoui Y, Ernst RK, Miao EA. Cytoplasmic LPS activates caspase-11: implications in TLR4-independent endotoxic shock. Science (2013) 341:1250–3. doi: 10.1126/science.1240988
88. Kayagaki N, Wong MT, Stowe IB, Ramani SR, Gonzalez LC, Akashi-Takamura S, et al. Noncanonical inflammasome activation by intracellular LPS independent of TLR4. Science (2013) 341:1246–9. doi: 10.1126/science.1240248
89. Shi J, Zhao Y, Wang Y, Gao W, Ding J, Li P, et al. Inflammatory caspases are innate immune receptors for intracellular LPS. Nature (2014) 514:187–92. doi: 10.1038/nature13683
90. Aglietti RA, Estevez A, Gupta A, Ramirez MG, Liu PS, Kayagaki N, et al. GsdmD p30 elicited by caspase-11 during pyroptosis forms pores in membranes. Proc Natl Acad Sci U.S.A. (2016) 113:7858–63. doi: 10.1073/pnas.1607769113
91. Shi J, Gao W, Shao F. Pyroptosis: Gasdermin-mediated programmed necrotic cell death. Trends Biochem Sci (2017) 42:245–54. doi: 10.1016/j.tibs.2016.10.004
92. Rühl S, Broz P. Caspase-11 activates a canonical NLRP3 inflammasome by promoting k(+) efflux. Eur J Immunol (2015) 45:2927–36. doi: 10.1002/eji.201545772
93. Baker PJ, Boucher D, Bierschenk D, Tebartz C, Whitney PG, D'Silva DB, et al. NLRP3 inflammasome activation downstream of cytoplasmic LPS recognition by both caspase-4 and caspase-5. Eur J Immunol (2015) 45:2918–26. doi: 10.1002/eji.201545655
94. Knodler LA, Crowley SM, Sham HP, Yang H, Wrande M, Ma C, et al. Noncanonical inflammasome activation of caspase-4/caspase-11 mediates epithelial defenses against enteric bacterial pathogens. Cell Host Microbe (2014) 16:249–56. doi: 10.1016/j.chom.2014.07.002
95. Yang D, He Y, Muñoz-Planillo R, Liu Q, Núñez G. Caspase-11 requires the pannexin-1 channel and the purinergic P2X7 pore to mediate pyroptosis and endotoxic shock. Immunity (2015) 43:923–32. doi: 10.1016/j.immuni.2015.10.009
96. Wandel MP, Kim BH, Park ES, Boyle KB, Nayak K, Lagrange B, et al. Guanylate-binding proteins convert cytosolic bacteria into caspase-4 signaling platforms. Nat Immunol (2020) 21:880–91. doi: 10.1038/s41590-020-0697-2
97. Santos JC, Broz P. Sensing of invading pathogens by GBPs: At the crossroads between cell-autonomous and innate immunity. J Leukoc Biol (2018) 104:729–35. doi: 10.1002/JLB.4MR0118-038R
98. Rogers C, Erkes DA, Nardone A, Aplin AE, Fernandes-Alnemri T, Alnemri ES. Gasdermin pores permeabilize mitochondria to augment caspase-3 activation during apoptosis and inflammasome activation. Nat Commun (2019) 10:1689. doi: 10.1038/s41467-019-09397-2
99. Zhang JY, Zhou B, Sun RY, Ai YL, Cheng K, Li FN, et al. The metabolite α-KG induces GSDMC-dependent pyroptosis through death receptor 6-activated caspase-8. Cell Res (2021) 31:980–97. doi: 10.1038/s41422-021-00506-9
100. Jiang S, Gu H, Zhao Y, Sun L. Teleost gasdermin e is cleaved by caspase 1, 3, and 7 and induces pyroptosis. J Immunol (2019) 203:1369–82. doi: 10.4049/jimmunol.1900383
101. Zhang Z, Zhang Y, Xia S, Kong Q, Li S, Liu X, et al. Gasdermin e suppresses tumour growth by activating anti-tumour immunity. Nature (2020) 579:415–20. doi: 10.1038/s41586-020-2071-9
102. Liu Y, Fang Y, Chen X, Wang Z, Liang X, Zhang T, et al. Gasdermin e-mediated target cell pyroptosis by CAR T cells triggers cytokine release syndrome. Sci Immunol (2020) 5:eaax7969. doi: 10.1126/sciimmunol.aax7969
103. Zhou Z, He H, Wang K, Shi X, Wang Y, Su Y, et al. Granzyme a from cytotoxic lymphocytes cleaves GSDMB to trigger pyroptosis in target cells. Science (2020) 368:eaaz7548. doi: 10.1126/science.aaz7548
104. Schroder K, Tschopp J. The inflammasomes. Cell (2010) 140:821–32. doi: 10.1016/j.cell.2010.01.040
105. Chen X, Zhang D, Li Y, Wang W, Bei W, Guo J. NLRP3 inflammasome and IL-1β pathway in type 2 diabetes and atherosclerosis: Friend or foe? Pharmacol Res (2021) 173:105885. doi: 10.1016/j.phrs.2021.105885
106. Lu A, Magupalli VG, Ruan J, Yin Q, Atianand MK, Vos MR, et al. Unified polymerization mechanism for the assembly of ASC-dependent inflammasomes. Cell (2014) 156:1193–206. doi: 10.1016/j.cell.2014.02.008
107. Patel S. Danger-associated molecular patterns (DAMPs): the derivatives and triggers of inflammation. Curr Allergy Asthma Rep (2018) 18:63. doi: 10.1007/s11882-018-0817-3
108. Chen C, Ma X, Yang C, Nie W, Zhang J, Li H, et al. Hypoxia potentiates LPS-induced inflammatory response and increases cell death by promoting NLRP3 inflammasome activation in pancreatic β cells. Biochem Biophys Res Commun (2018) 495:2512–8. doi: 10.1016/j.bbrc.2017.12.134
109. Tessaro FHG, Ayala TS, Nolasco EL, Bella LM, Martins JO. Insulin influences LPS-induced TNF-α and IL-6 release through distinct pathways in mouse macrophages from different compartments. Cell Physiol Biochem (2017) 42:2093–104. doi: 10.1159/000479904
110. Nishikawa T, Araki E. Impact of mitochondrial ROS production in the pathogenesis of diabetes mellitus and its complications. Antioxid Redox Signal (2007) 9:343–53. doi: 10.1089/ars.2006.1458
111. Masters SL, Dunne A, Subramanian SL, Hull RL, Tannahill GM, Sharp FA, et al. Activation of the NLRP3 inflammasome by islet amyloid polypeptide provides a mechanism for enhanced IL-1β in type 2 diabetes. Nat Immunol (2010) 11:897–904. doi: 10.1038/ni.1935
112. Vandanmagsar B, Youm YH, Ravussin A, Galgani JE, Stadler K, Mynatt RL, et al. The NLRP3 inflammasome instigates obesity-induced inflammation and insulin resistance. Nat Med (2011) 17:179–88. doi: 10.1038/nm.2279
113. Zhang SY, Dong YQ, Wang P, Zhang X, Yan Y, Sun L, et al. Adipocyte-derived lysophosphatidylcholine activates adipocyte and adipose tissue macrophage nod-like receptor protein 3 inflammasomes mediating homocysteine-induced insulin resistance. EBioMedicine (2018) 31:202–16. doi: 10.1016/j.ebiom.2018.04.022
114. Lukens JR, Dixit VD, Kanneganti TD. Inflammasome activation in obesity-related inflammatory diseases and autoimmunity. Discovery Med (2011) 12:65–74.
115. Schroder K, Zhou R, Tschopp J. The NLRP3 inflammasome: a sensor for metabolic danger? Science (2010) 327:296–300. doi: 10.1126/science.1184003
116. Stienstra R, Joosten LA, Koenen T, van Tits B, van Diepen JA, van den Berg SA, et al. The inflammasome-mediated caspase-1 activation controls adipocyte differentiation and insulin sensitivity. Cell Metab (2010) 12:593–605. doi: 10.1016/j.cmet.2010.11.011
117. Zhou R, Tardivel A, Thorens B, Choi I, Tschopp J. Thioredoxin-interacting protein links oxidative stress to inflammasome activation. Nat Immunol (2010) 11:136–40. doi: 10.1038/ni.1831
118. Wen H, Gris D, Lei Y, Jha S, Zhang L, Huang MT, et al. Fatty acid-induced NLRP3-ASC inflammasome activation interferes with insulin signaling. Nat Immunol (2011) 12:408–15. doi: 10.1038/ni.2022
119. Lee HM, Kim JJ, Kim HJ, Shong M, Ku BJ, Jo EK. Upregulated NLRP3 inflammasome activation in patients with type 2 diabetes. Diabetes (2013) 62:194–204. doi: 10.2337/db12-0420
120. Lin CF, Kuo YT, Chen TY, Chien CT. Quercetin-rich guava (Psidium guajava) juice in combination with trehalose reduces autophagy, apoptosis and pyroptosis formation in the kidney and pancreas of type II diabetic rats. Molecules (2016) 21:334. doi: 10.3390/molecules21030334
121. Yuan J, Li S, Peng H, Ma Y, Li L, Fu L, et al. Artesunate protects pancreatic β-cells from streptozotocin-induced diabetes via inhibition of the NLRP3/caspase-1/GSDMD pathway. Gen Comp Endocrinol (2022) 326:114068. doi: 10.1016/j.ygcen.2022.114068
122. Zuo Y, Chen L, Gu H, He X, Ye Z, Wang Z, et al. GSDMD-mediated pyroptosis: a critical mechanism of diabetic nephropathy. Expert Rev Mol Med (2021) 23:e23. doi: 10.1017/erm.2021.27
123. Lu Y, Lu Y, Meng J, Wang Z. Pyroptosis and its regulation in diabetic cardiomyopathy. Front Physiol (2021) 12:791848. doi: 10.3389/fphys.2021.791848
124. Song D, Li M, Yu X, Wang Y, Fan J, Yang W, et al. The molecular pathways of pyroptosis in atherosclerosis. Front Cell Dev Biol (2022) 10:824165. doi: 10.3389/fcell.2022.824165
125. Xu J, Cai S, Zhao J, Xu K, Ji H, Wu C, et al. Advances in the relationship between pyroptosis and diabetic neuropathy. Front Cell Dev Biol (2021) 9:753660. doi: 10.3389/fcell.2021.753660
126. Umanath K, Lewis JB. Update on diabetic nephropathy: Core curriculum 2018. Am J Kidney Dis (2018) 71:884–95. doi: 10.1053/j.ajkd.2017.10.026
127. Shahzad K, Bock F, Dong W, Wang H, Kopf S, Kohli S, et al. Nlrp3-inflammasome activation in non-myeloid-derived cells aggravates diabetic nephropathy. Kidney Int (2015) 87:74–84. doi: 10.1038/ki.2014.271
128. Al Mamun A, Ara Mimi A, Wu Y, Zaeem M, Abdul Aziz M, Aktar Suchi S, et al. Pyroptosis in diabetic nephropathy. Clin Chim Acta (2021) 523:131–43. doi: 10.1016/j.cca.2021.09.003
129. Zhan JF, Huang HW, Huang C, Hu LL, Xu WW. Long non-coding RNA NEAT1 regulates pyroptosis in diabetic nephropathy via mediating the miR-34c/NLRP3 axis. Kidney Blood Press Res (2020) 45:589–602. doi: 10.1159/000508372
130. An X, Zhang Y, Cao Y, Chen J, Qin H, Yang L. Punicalagin protects diabetic nephropathy by inhibiting pyroptosis based on TXNIP/NLRP3 pathway. Nutrients (2020) 12:1516. doi: 10.3390/nu12051516
131. Li F, Chen Y, Li Y, Huang M, Zhao W. Geniposide alleviates diabetic nephropathy of mice through AMPK/SIRT1/NF-κB pathway. Eur J Pharmacol (2020) 886:173449. doi: 10.1016/j.ejphar.2020.173449
132. Wen S, Deng F, Li L, Xu L, Li X, Fan Q. VX-765 ameliorates renal injury and fibrosis in diabetes by regulating caspase-1-mediated pyroptosis and inflammation. J Diabetes Investig (2022) 13:22–33. doi: 10.1111/jdi.13660
133. Gao C, Wang B, Chen Q, Wang M, Fei X, Zhao N. Serum exosomes from diabetic kidney disease patients promote pyroptosis and oxidative stress through the miR-4449/HIC1 pathway. Nutr Diabetes (2021) 11:33. doi: 10.1038/s41387-021-00175-y
134. Ke R, Wang Y, Hong S, Xiao L. Endoplasmic reticulum stress related factor IRE1α regulates TXNIP/NLRP3-mediated pyroptosis in diabetic nephropathy. Exp Cell Res (2020) 396:112293. doi: 10.1016/j.yexcr.2020.112293
135. Gao Y, Ma Y, Xie D, Jiang H. ManNAc protects against podocyte pyroptosis via inhibiting mitochondrial damage and ROS/NLRP3 signaling pathway in diabetic kidney injury model. Int Immunopharmacol (2022) 107:108711. doi: 10.1016/j.intimp.2022.108711
136. El-Lateef AEA, El-Shemi AGA, Alhammady MS, Yuan R, Zhang Y. LncRNA NEAT2 modulates pyroptosis of renal tubular cells induced by high glucose in diabetic nephropathy (DN) by via miR-206 regulation. Biochem Genet (2022) 60:1733–47. doi: 10.1007/s10528-021-10164-6
137. Zhang Z, Shao X, Jiang N, Mou S, Gu L, Li S, et al. Caspase-11-mediated tubular epithelial pyroptosis underlies contrast-induced acute kidney injury. Cell Death Dis (2018) 9:983. doi: 10.1038/s41419-018-1023-x
138. Garg P. A review of podocyte biology. Am J Nephrol (2018) 47 Suppl 1:3–13. doi: 10.1159/000481633
139. Dai H, Liu Q, Liu B. Research progress on mechanism of podocyte depletion in diabetic nephropathy. J Diabetes Res (2017) 2017:2615286. doi: 10.1155/2017/2615286
140. Cheng Q, Pan J, Zhou ZL, Yin F, Xie HY, Chen PP, et al. Caspase-11/4 and gasdermin d-mediated pyroptosis contributes to podocyte injury in mouse diabetic nephropathy. Acta Pharmacol Sin (2021) 42:954–63. doi: 10.1038/s41401-020-00525-z
141. Liu Y, Xu Z, Ma F, Jia Y, Wang G. Knockdown of TLR4 attenuates high glucose-induced podocyte injury via the NALP3/ASC/Caspase-1 signaling pathway. BioMed Pharmacother (2018) 107:1393–401. doi: 10.1016/j.biopha.2018.08.134
142. Abais JM, Zhang C, Xia M, Liu Q, Gehr TW, Boini KM, et al. NADPH oxidase-mediated triggering of inflammasome activation in mouse podocytes and glomeruli during hyperhomocysteinemia. Antioxid Redox Signal (2013) 18:1537–48. doi: 10.1089/ars.2012.4666
143. Hong J, Li G, Zhang Q, Ritter J, Li W, Li PL. D-ribose induces podocyte NLRP3 inflammasome activation and glomerular injury via AGEs/RAGE pathway. Front Cell Dev Biol (2019) 7:259. doi: 10.3389/fcell.2019.00259
144. Yoon MS. The role of mammalian target of rapamycin (mTOR) in insulin signaling. Nutrients (2017) 9:1176. doi: 10.3390/nu9111176
145. Cosin-Roger J, Simmen S, Melhem H, Atrott K, Frey-Wagner I, Hausmann M, et al. Hypoxia ameliorates intestinal inflammation through NLRP3/mTOR downregulation and autophagy activation. Nat Commun (2017) 8:98. doi: 10.1038/s41467-017-00213-3
146. Jia X, Liu B, Bao L, Lv Q, Li F, Li H, et al. Delayed oseltamivir plus sirolimus treatment attenuates H1N1 virus-induced severe lung injury correlated with repressed NLRP3 inflammasome activation and inflammatory cell infiltration. PloS Pathog (2018) 14:e1007428. doi: 10.1371/journal.ppat.1007428
147. Wang T, Gao Y, Yue R, Wang X, Shi Y, Xu J, et al. Ginsenoside Rg1 alleviates podocyte injury induced by hyperlipidemia via targeting the mTOR/NF-κB/NLRP3 axis. Evid Based Complement Alternat Med (2020) 2020:2735714. doi: 10.1155/2020/2735714
148. Ding X, Jing N, Shen A, Guo F, Song Y, Pan M, et al. MiR-21-5p in macrophage-derived extracellular vesicles affects podocyte pyroptosis in diabetic nephropathy by regulating A20. J Endocrinol Invest (2021) 44:1175–84. doi: 10.1007/s40618-020-01401-7
149. Wang H, Lv D, Jiang S, Hou Q, Zhang L, Li S, et al. Complement induces podocyte pyroptosis in membranous nephropathy by mediating mitochondrial dysfunction. Cell Death Dis (2022) 13:281. doi: 10.1038/s41419-022-04737-5
150. Wang MZ, Wang J, Cao DW, Tu Y, Liu BH, Yuan CC, et al. Fucoidan alleviates renal fibrosis in diabetic kidney disease via inhibition of NLRP3 inflammasome-mediated podocyte pyroptosis. Front Pharmacol (2022) 13:790937. doi: 10.3389/fphar.2022.790937
151. Xu X, Zhang L, Hua F, Zhang C, Zhang C, Mi X, et al. FOXM1-activated SIRT4 inhibits NF-κB signaling and NLRP3 inflammasome to alleviate kidney injury and podocyte pyroptosis in diabetic nephropathy. Exp Cell Res (2021) 408:112863. doi: 10.1016/j.yexcr.2021.112863
152. Chen SJ, Lv LL, Liu BC, Tang RN. Crosstalk between tubular epithelial cells and glomerular endothelial cells in diabetic kidney disease. Cell Prolif (2020) 53:e12763. doi: 10.1111/cpr.12763
153. Xie C, Wu W, Tang A, Luo N, Tan Y. lncRNA GAS5/miR-452-5p reduces oxidative stress and pyroptosis of high-Glucose-Stimulated renal tubular cells. Diabetes Metab Syndr Obes (2019) 12:2609–17. doi: 10.2147/DMSO.S228654
154. Zhu B, Cheng X, Jiang Y, Cheng M, Chen L, Bao J, et al. Silencing of KCNQ1OT1 decreases oxidative stress and pyroptosis of renal tubular epithelial cells. Diabetes Metab Syndr Obes (2020) 13:365–75. doi: 10.2147/DMSO.S225791
155. Wang Y, Zhu X, Yuan S, Wen S, Liu X, Wang C, et al. TLR4/NF-κB signaling induces GSDMD-related pyroptosis in tubular cells in diabetic kidney disease. Front Endocrinol (Lausanne) (2019) 10:603. doi: 10.3389/fendo.2019.00603
156. Wang J, Zhao SM. LncRNA-antisense non-coding RNA in the INK4 locus promotes pyroptosis via miR-497/thioredoxin-interacting protein axis in diabetic nephropathy. Life Sci (2021) 264:118728. doi: 10.1016/j.lfs.2020.118728
157. Meng C, Gu C, He S, Su T, Lhamo T, Draga D, et al. Pyroptosis in the retinal neurovascular unit: New insights into diabetic retinopathy. Front Immunol (2021) 12:763092. doi: 10.3389/fimmu.2021.763092
158. Yu X, Ma X, Lin W, Xu Q, Zhou H, Kuang H. Long noncoding RNA MIAT regulates primary human retinal pericyte pyroptosis by modulating miR-342-3p targeting of CASP1 in diabetic retinopathy. Exp Eye Res (2021) 202:108300. doi: 10.1016/j.exer.2020.108300
159. Loukovaara S, Piippo N, Kinnunen K, Hytti M, Kaarniranta K, Kauppinen A. NLRP3 inflammasome activation is associated with proliferative diabetic retinopathy. Acta Ophthalmol (2017) 95:803–8. doi: 10.1111/aos.13427
160. Yin Y, Chen F, Wang W, Wang H, Zhang X. Resolvin D1 inhibits inflammatory response in STZ-induced diabetic retinopathy rats: Possible involvement of NLRP3 inflammasome and NF-κB signaling pathway. Mol Vis (2017) 23:242–50.
161. Gan J, Huang M, Lan G, Liu L, Xu F. High glucose induces the loss of retinal pericytes partly via NLRP3-Caspase-1-GSDMD-Mediated pyroptosis. BioMed Res Int (2020) 2020:4510628. doi: 10.1155/2020/4510628
162. Gu C, Draga D, Zhou C, Su T, Zou C, Gu Q, et al. miR-590-3p inhibits pyroptosis in diabetic retinopathy by targeting NLRP1 and inactivating the NOX4 signaling pathway. Invest Ophthalmol Vis Sci (2019) 60:4215–23. doi: 10.1167/iovs.19-27825
163. Viringipurampeer IA, Metcalfe AL, Bashar AE, Sivak O, Yanai A, Mohammadi Z, et al. NLRP3 inflammasome activation drives bystander cone photoreceptor cell death in a P23H rhodopsin model of retinal degeneration. Hum Mol Genet (2016) 25:1501–16. doi: 10.1093/hmg/ddw029
164. Zha X, Xi X, Fan X, Ma M, Zhang Y, Yang Y. Overexpression of METTL3 attenuates high-glucose induced RPE cell pyroptosis by regulating miR-25-3p/PTEN/Akt signaling cascade through DGCR8. Aging (Albany NY) (2020) 12:8137–50. doi: 10.18632/aging.103130
165. Sun Q, Wang C, Yan B, Shi X, Shi Y, Qu L, et al. Jinmaitong ameliorates diabetic peripheral neuropathy through suppressing TXNIP/NLRP3 inflammasome activation in the streptozotocin-induced diabetic rat model. Diabetes Metab Syndr Obes (2019) 12:2145–55. doi: 10.2147/DMSO.S223842
166. Papa G, Degano C, Iurato MP, Licciardello C, Maiorana R, Finocchiaro C. Macrovascular complication phenotypes in type 2 diabetic patients. Cardiovasc Diabetol (2013) 12:20. doi: 10.1186/1475-2840-12-20
167. Bai Y, Mu Q, Bao X, Zuo J, Fang X, Hua J, et al. Targeting NLRP3 inflammasome in the treatment of diabetes and diabetic complications: Role of natural compounds from herbal medicine. Aging Dis (2021) 12:1587–604. doi: 10.14336/AD.2021.0318
168. Zeng C, Wang R, Tan H. Role of pyroptosis in cardiovascular diseases and its therapeutic implications. Int J Biol Sci (2019) 15:1345–57. doi: 10.7150/ijbs.33568
169. An Q, Hu Q, Wang B, Cui W, Wu F, Ding Y. Oleanolic acid alleviates diabetic rat carotid artery injury through the inhibition of NLRP3 inflammasome signaling pathways. Mol Med Rep (2017) 16:8413–9. doi: 10.3892/mmr.2017.7594
170. Ma Q, Yang Q, Chen J, Yu C, Zhang L, Zhou W, et al. Salvianolic acid a ameliorates early-stage atherosclerosis development by inhibiting NLRP3 inflammasome activation in zucker diabetic fatty rats. Molecules (2020) 25:1089. doi: 10.3390/molecules25051089
171. Song J, Li J, Hou F, Wang X, Liu B. Mangiferin inhibits endoplasmic reticulum stress-associated thioredoxin-interacting protein/NLRP3 inflammasome activation with regulation of AMPK in endothelial cells. Metabolism (2015) 64:428–37. doi: 10.1016/j.metabol.2014.11.008
172. Chen TC, Yen CK, Lu YC, Shi CS, Hsieh RZ, Chang SF, et al. The antagonism of 6-shogaol in high-glucose-activated NLRP3 inflammasome and consequent calcification of human artery smooth muscle cells. Cell Biosci (2020) 10:5. doi: 10.1186/s13578-019-0372-1
173. Oh S, Son M, Park CH, Jang JT, Son KH, Byun K. The reducing effects of pyrogallol-Phloroglucinol-6,6-Bieckol on high-fat diet-induced pyroptosis in endothelial and vascular smooth muscle cells of mice aortas. Mar Drugs (2020) 18:648. doi: 10.3390/md18120648
174. Murtaza G, Virk HUH, Khalid M, Lavie CJ, Ventura H, Mukherjee D, et al. Diabetic cardiomyopathy - a comprehensive updated review. Prog Cardiovasc Dis (2019) 62:315–26. doi: 10.1016/j.pcad.2019.03.003
175. Xie Y, Huang Y, Ling X, Qin H, Wang M, Luo B. Chemerin/CMKLR1 axis promotes inflammation and pyroptosis by activating NLRP3 inflammasome in diabetic cardiomyopathy rat. Front Physiol (2020) 11:381. doi: 10.3389/fphys.2020.00381
176. Zhou W, Chen C, Chen Z, Liu L, Jiang J, Wu Z, et al. NLRP3: A novel mediator in cardiovascular disease. J Immunol Res (2018) 2018:5702103. doi: 10.1155/2018/5702103
177. Luo B, Li B, Wang W, Liu X, Xia Y, Zhang C, et al. NLRP3 gene silencing ameliorates diabetic cardiomyopathy in a type 2 diabetes rat model. PloS One (2014) 9:e104771. doi: 10.1371/journal.pone.0104771
178. Qiu Z, Lei S, Zhao B, Wu Y, Su W, Liu M, et al. NLRP3 inflammasome activation-mediated pyroptosis aggravates myocardial Ischemia/Reperfusion injury in diabetic rats. Oxid Med Cell Longev (2017) 2017:9743280. doi: 10.1155/2017/9743280
179. Zhaolin Z, Guohua L, Shiyuan W, Zuo W. Role of pyroptosis in cardiovascular disease. Cell Prolif (2019) 52:e12563. doi: 10.1111/cpr.12563
180. Chen Y, Hua Y, Li X, Arslan IM, Zhang W, Meng G. Distinct types of cell death and the implication in diabetic cardiomyopathy. Front Pharmacol (2020) 11:42. doi: 10.3389/fphar.2020.00042
181. Wei H, Bu R, Yang Q, Jia J, Li T, Wang Q, et al. Exendin-4 protects against hyperglycemia-induced cardiomyocyte pyroptosis via the AMPK-TXNIP pathway. J Diabetes Res (2019) 2019:8905917. doi: 10.1155/2019/8905917
182. Singla DK, Johnson TA, Tavakoli Dargani Z. Exosome treatment enhances anti-inflammatory M2 macrophages and reduces inflammation-induced pyroptosis in doxorubicin-induced cardiomyopathy. Cells (2019) 8:1224. doi: 10.3390/cells8101224
183. Li S, Liu R, Xue M, Qiao Y, Chen Y, Long G, et al. Spleen tyrosine kinase−induced JNK−dependent NLRP3 activation is involved in diabetic cardiomyopathy. Int J Mol Med (2019) 43:2481–90. doi: 10.3892/ijmm.2019.4148
184. Zhang H, Chen X, Zong B, Yuan H, Wang Z, Wei Y, et al. Gypenosides improve diabetic cardiomyopathy by inhibiting ROS-mediated NLRP3 inflammasome activation. J Cell Mol Med (2018) 22:4437–48. doi: 10.1111/jcmm.13743
185. Li X, Du N, Zhang Q, Li J, Chen X, Liu X, et al. MicroRNA-30d regulates cardiomyocyte pyroptosis by directly targeting foxo3a in diabetic cardiomyopathy. Cell Death Dis (2014) 5:e1479. doi: 10.1038/cddis.2014.430
186. Yang F, Qin Y, Lv J, Wang Y, Che H, Chen X, et al. Silencing long non-coding RNA Kcnq1ot1 alleviates pyroptosis and fibrosis in diabetic cardiomyopathy. Cell Death Dis (2018) 9:1000. doi: 10.1038/s41419-018-1029-4
187. Jeyabal P, Thandavarayan RA, Joladarashi D, Suresh Babu S, Krishnamurthy S, Bhimaraj A, et al. MicroRNA-9 inhibits hyperglycemia-induced pyroptosis in human ventricular cardiomyocytes by targeting ELAVL1. Biochem Biophys Res Commun (2016) 471:423–9. doi: 10.1016/j.bbrc.2016.02.065
188. Nazir S, Gadi I, Al-Dabet MM, Elwakiel A, Kohli S, Ghosh S, et al. Cytoprotective activated protein c averts Nlrp3 inflammasome-induced ischemia-reperfusion injury via mTORC1 inhibition. Blood (2017) 130:2664–77. doi: 10.1182/blood-2017-05-782102
189. Li X, He X, Wang H, Li M, Huang S, Chen G, et al. Loss of AZIN2 splice variant facilitates endogenous cardiac regeneration. Cardiovasc Res (2018) 114:1642–55. doi: 10.1093/cvr/cvy075
190. Zhang X, Fu Y, Li H, Shen L, Chang Q, Pan L, et al. H3 relaxin inhibits the collagen synthesis via ROS- and P2X7R-mediated NLRP3 inflammasome activation in cardiac fibroblasts under high glucose. J Cell Mol Med (2018) 22:1816–25. doi: 10.1111/jcmm.13464
191. Shi P, Zhao XD, Shi KH, Ding XS, Tao H. MiR-21-3p triggers cardiac fibroblasts pyroptosis in diabetic cardiac fibrosis via inhibiting androgen receptor. Exp Cell Res (2021) 399:112464. doi: 10.1016/j.yexcr.2020.112464
192. Ren L, Chen X, Nie B, Qu H, Ju J, Bai Y. Ranolazine inhibits pyroptosis via regulation of miR-135b in the treatment of diabetic cardiac fibrosis. Front Mol Biosci (2022) 9:806966. doi: 10.3389/fmolb.2022.806966
193. Chen R, Shi J, Yin Q, Li X, Sheng Y, Han J, et al. Morphological and pathological characteristics of brain in diabetic encephalopathy. J Alzheimers Dis (2018) 65:15–28. doi: 10.3233/JAD-180314
194. Huang L, You J, Yao Y, Xie M. High glucose induces pyroptosis of retinal microglia through NLPR3 inflammasome signaling. Arq Bras Oftalmol (2021) 84:67–73. doi: 10.5935/0004-2749.20210010
195. Ye L, Li G, Goebel A, Raju AV, Kong F, Lv Y, et al. Caspase-11-mediated enteric neuronal pyroptosis underlies Western diet-induced colonic dysmotility. J Clin Invest (2020) 130:3621–36. doi: 10.1172/JCI130176
196. Hong P, Li FX, Gu RN, Fang YY, Lai LY, Wang YW, et al. Inhibition of NLRP3 inflammasome ameliorates cerebral ischemia-reperfusion injury in diabetic mice. Neural Plast (2018) 2018:9163521. doi: 10.1155/2018/9163521
197. Wang LQ, Zheng YY, Zhou HJ, Zhang XX, Wu P, Zhu SM. LncRNA-fendrr protects against the ubiquitination and degradation of NLRC4 protein through HERC2 to regulate the pyroptosis of microglia. Mol Med (2021) 27:39. doi: 10.1186/s10020-021-00299-y
198. Li DX, Wang CN, Wang Y, Ye CL, Jiang L, Zhu XY, et al. NLRP3 inflammasome-dependent pyroptosis and apoptosis in hippocampus neurons mediates depressive-like behavior in diabetic mice. Behav Brain Res (2020) 391:112684. doi: 10.1016/j.bbr.2020.112684
199. Che H, Li H, Li Y, Wang YQ, Yang ZY, Wang RL, et al. Melatonin exerts neuroprotective effects by inhibiting neuronal pyroptosis and autophagy in STZ-induced diabetic mice. FASEB J (2020) 34:14042–54. doi: 10.1096/fj.202001328R
200. Sun Q, Wang C, Yan B, Shi HX, Shi Y, Qu L, et al. Expressions of thioredoxin interacting Protein/Nucleotide-binding oligomerization domain-like receptor protein 3 inflammasome in the sciatic nerve of streptozotocin-induced diabetic rats. Zhongguo Yi Xue Ke Xue Yuan Xue Bao (2019) 41:799–805. doi: 10.3881/j.issn.1000-503X.11468
201. Cheng YC, Chu LW, Chen JY, Hsieh SL, Chang YC, Dai ZK, et al. Loganin attenuates high glucose-induced schwann cells pyroptosis by inhibiting ROS generation and NLRP3 inflammasome activation. Cells (2020) 9:1948. doi: 10.3390/cells9091948
202. Li R, Wang B, Wu C, Li D, Wu Y, Ye L, et al. Acidic fibroblast growth factor attenuates type 2 diabetes-induced demyelination via suppressing oxidative stress damage. Cell Death Dis (2021) 12:107. doi: 10.1038/s41419-021-03407-2
203. Xu L, Lin X, Guan M, Zeng Y, Liu Y. Verapamil attenuated prediabetic neuropathy in high-fat diet-fed mice through inhibiting TXNIP-mediated apoptosis and inflammation. Oxid Med Cell Longev (2019) 2019:1896041. doi: 10.1155/2019/1896041
204. Lebeaupin C, Proics E, de Bieville CH, Rousseau D, Bonnafous S, Patouraux S, et al. ER stress induces NLRP3 inflammasome activation and hepatocyte death. Cell Death Dis (2015) 6:e1879. doi: 10.1038/cddis.2015.248
205. Wree A, Eguchi A, McGeough MD, Pena CA, Johnson CD, Canbay A, et al. NLRP3 inflammasome activation results in hepatocyte pyroptosis, liver inflammation, and fibrosis in mice. Hepatology (2014) 59:898–910. doi: 10.1002/hep.26592
206. Robert S, Gicquel T, Bodin A, Lagente V, Boichot E. Characterization of the MMP/TIMP imbalance and collagen production induced by IL-1β or TNF-α release from human hepatic stellate cells. PloS One (2016) 11:e0153118. doi: 10.1371/journal.pone.0153118
207. Gaul S, Leszczynska A, Alegre F, Kaufmann B, Johnson CD, Adams LA, et al. Hepatocyte pyroptosis and release of inflammasome particles induce stellate cell activation and liver fibrosis. J Hepatol (2021) 74:156–67. doi: 10.1016/j.jhep.2020.07.041
208. Wree A, McGeough MD, Inzaugarat ME, Eguchi A, Schuster S, Johnson CD, et al. NLRP3 inflammasome driven liver injury and fibrosis: Roles of IL-17 and TNF in mice. Hepatology (2018) 67:736–49. doi: 10.1002/hep.29523
209. Yang F, Qin Y, Wang Y, Meng S, Xian H, Che H, et al. Metformin inhibits the NLRP3 inflammasome via AMPK/mTOR-dependent effects in diabetic cardiomyopathy. Int J Biol Sci (2019) 15:1010–9. doi: 10.7150/ijbs.29680
210. Zhang J, Huang L, Shi X, Yang L, Hua F, Ma J, et al. Metformin protects against myocardial ischemia-reperfusion injury and cell pyroptosis via AMPK/NLRP3 inflammasome pathway. Aging (Albany NY) (2020) 12:24270–87. doi: 10.18632/aging.202143
211. Zou R, Nie C, Pan S, Wang B, Hong X, Xi S, et al. Co-Administration of hydrogen and metformin exerts cardioprotective effects by inhibiting pyroptosis and fibrosis in diabetic cardiomyopathy. Free Radic Biol Med (2022) 183:35–50. doi: 10.1016/j.freeradbiomed.2022.03.010
212. Song S, Guo R, Mehmood A, Zhang L, Yin B, Yuan C, et al. Liraglutide attenuate central nervous inflammation and demyelination through AMPK and pyroptosis-related NLRP3 pathway. CNS Neurosci Ther (2022) 28:422–34. doi: 10.1111/cns.13791
213. Chen A, Chen Z, Xia Y, Lu D, Yang X, Sun A, et al. Liraglutide attenuates NLRP3 inflammasome-dependent pyroptosis via regulating SIRT1/NOX4/ROS pathway in H9c2 cells. Biochem Biophys Res Commun (2018) 499:267–72. doi: 10.1016/j.bbrc.2018.03.142
214. Yu X, Hao M, Liu Y, Ma X, Lin W, Xu Q, et al. Liraglutide ameliorates non-alcoholic steatohepatitis by inhibiting NLRP3 inflammasome and pyroptosis activation via mitophagy. Eur J Pharmacol (2019) 864:172715. doi: 10.1016/j.ejphar.2019.172715
215. Liu Y, Wang DW, Wang D, Duan BH, Kuang HY. Exenatide attenuates non-alcoholic steatohepatitis by inhibiting the pyroptosis signaling pathway. Front Endocrinol (Lausanne) (2021) 12:663039. doi: 10.3389/fendo.2021.663039
216. Ye Y, Bajaj M, Yang HC, Perez-Polo JR, Birnbaum Y. SGLT-2 inhibition with dapagliflozin reduces the activation of the Nlrp3/ASC inflammasome and attenuates the development of diabetic cardiomyopathy in mice with type 2 diabetes. Further Augmentation Effects Saxagliptin DPP4 Inhibitor. Cardiovasc Drugs Ther (2017) 31:119–32. doi: 10.1007/s10557-017-6725-2
217. Zhu Y, Zhu C, Yang H, Deng J, Fan D. Protective effect of ginsenoside Rg5 against kidney injury via inhibition of NLRP3 inflammasome activation and the MAPK signaling pathway in high-fat diet/streptozotocin-induced diabetic mice. Pharmacol Res (2020) 155:104746. doi: 10.1016/j.phrs.2020.104746
218. Han W, Ma Q, Liu Y, Wu W, Tu Y, Huang L, et al. Huangkui capsule alleviates renal tubular epithelial-mesenchymal transition in diabetic nephropathy via inhibiting NLRP3 inflammasome activation and TLR4/NF-κB signaling. Phytomedicine (2019) 57:203–14. doi: 10.1016/j.phymed.2018.12.021
219. Zheng T, Yang X, Li W, Wang Q, Chen L, Wu D, et al. Salidroside attenuates high-fat diet-induced nonalcoholic fatty liver disease via AMPK-dependent TXNIP/NLRP3 pathway. Oxid Med Cell Longev (2018) 2018:8597897. doi: 10.1155/2018/8597897
220. Li S, Yang H, Chen X. Protective effects of sulforaphane on diabetic retinopathy: activation of the Nrf2 pathway and inhibition of NLRP3 inflammasome formation. Exp Anim (2019) 68:221–31. doi: 10.1538/expanim.18-0146
221. Zhang Y, Liu X, Bai X, Lin Y, Li Z, Fu J, et al. Melatonin prevents endothelial cell pyroptosis via regulation of long noncoding RNA MEG3/miR-223/NLRP3 axis. J Pineal Res (2018) 64:12449. doi: 10.1111/jpi.12449
222. Lu J, Xu L, Zeng Z, Xue C, Li J, Chen X, et al. Normothermic ex vivo heart perfusion combined with melatonin enhances myocardial protection in rat donation after circulatory death hearts via inhibiting NLRP3 inflammasome-mediated pyroptosis. Front Cell Dev Biol (2021) 9:733183. doi: 10.3389/fcell.2021.733183
223. Yang K, Liu J, Zhang X, Ren Z, Gao L, Wang Y, et al. H3 relaxin alleviates migration, apoptosis and pyroptosis through P2X7R-mediated nucleotide binding oligomerization domain-like receptor protein 3 inflammasome activation in retinopathy induced by hyperglycemia. Front Pharmacol (2020) 11:603689. doi: 10.3389/fphar.2020.603689
224. Zhang X, Pan L, Yang K, Fu Y, Liu Y, Chi J, et al. H3 relaxin protects against myocardial injury in experimental diabetic cardiomyopathy by inhibiting myocardial apoptosis, fibrosis and inflammation. Cell Physiol Biochem (2017) 43:1311–24. doi: 10.1159/000481843
225. Gu C, Zhang H, Li Q, Zhao S, Gao Y. MiR-192 attenuates high glucose-induced pyroptosis in retinal pigment epithelial cells via inflammasome modulation. Bioengineered (2022) 13:10362–72. doi: 10.1080/21655979.2022.2044734
226. Li X, Bai C, Wang H, Wan T, Li Y. LncRNA MEG3 regulates autophagy and pyroptosis via FOXO1 in pancreatic β-cells. Cell Signal (2022) 92:110247. doi: 10.1016/j.cellsig.2022.110247
Keywords: type 2 diabetes, pyroptosis, NLRP3 inflammasome, complications, therapy
Citation: Li X, Xiao G-Y, Guo T, Song Y-J and Li Q-M (2022) Potential therapeutic role of pyroptosis mediated by the NLRP3 inflammasome in type 2 diabetes and its complications. Front. Endocrinol. 13:986565. doi: 10.3389/fendo.2022.986565
Received: 05 July 2022; Accepted: 13 October 2022;
Published: 27 October 2022.
Edited by:
Kamaldeep Paul, Thapar Institute of Engineering & Technology, IndiaReviewed by:
Zhaoyu Lin, Nanjing University, ChinaKaifeng Guo, Fudan University, China
Weitao Yan, Central South University, China
Copyright © 2022 Li, Xiao, Guo, Song and Li. This is an open-access article distributed under the terms of the Creative Commons Attribution License (CC BY). The use, distribution or reproduction in other forums is permitted, provided the original author(s) and the copyright owner(s) are credited and that the original publication in this journal is cited, in accordance with accepted academic practice. No use, distribution or reproduction is permitted which does not comply with these terms.
*Correspondence: Qiu-Mei Li, lqm5800@sina.com